Research Article
The Influence of Sleep on Brain Health
Samer Younes, Department of Pharmacy, Tartous University, Syria
Received Date:March 25, 2024; Published Date:April 05, 2024
Abstract
Sleep plays a crucial role in maintaining brain health and cognitive function. It is well-known that disrupted sleep is a common symptom of Alzheimer’s disease (AD). However, emerging evidence suggests that even suboptimal sleep can increase the risk of developing AD. The deacetylase Sirtuin 1 (Sirt 1), which is encoded by the SIRT1 gene, has been found to influence sleep by affecting wake-sleep neurotransmitters and somnogens. Both animal and human studies have provided support for a complex relationship between sleep, Sirt 1/SIRT1, and AD. Various hypotheses have been proposed to explain the significant impact of Sirt 1/SIRT1 on neurons involved in promoting wakefulness and sleep, as well as their associated mechanisms and neurotransmitters. However, there is a lack of research investigating the interaction between sleep and Sirt 1/SIRT1 as a key component of sleep regulation in relation to AD pathology. In this review, we aim to explore the potential association between Sirt 1/SIRT1, sleep, and the development of AD. Considering that sleep is a modifiable risk factor for AD and recent studies suggest that the activation of Sirt 1/SIRT1 can be influenced by lifestyle and dietary interventions, further research in this area is necessary to investigate its potential as a target for the prevention and treatment of AD.
Keywords:Brain Health; Slow Wave Sleep; NREM Sleep
Introduction
Insufficient sleep or poor sleep quality has been linked to a wide range of negative health outcomes and subsequent economic burdens [1]. Research has shown that sleep plays a crucial role in brain health and the maintenance of cognitive abilities by regulating neuroplasticity, information processing, and memory consolidation [2]. Older adults often experience suboptimal sleep, characterized by difficulties in falling and staying asleep, frequent awakenings, and a decrease in restorative slow-wave sleep and rapid eye movement (REM) sleep [3]. The prevalence of these sleep disturbances tends to increase with age and can be attributed to various factors such as changes in sleep behavior due to aging, stress, anxiety, medication use, underlying diseases, or a combination of these factors [4].
A growing body of literature has focused on the relationship between sleep disturbances and neurodegenerative diseases. Patients with Alzheimer’s disease (AD) often exhibit clinical symptoms of sleep abnormalities, with increased fragmentation being one of the earliest reported symptoms or complaints [5]. Electroencephalogram (EEG) recordings in AD patients have shown a significant reduction in slow-wave sleep and REM sleep, as well as disruptions in arousal in response to external stimuli [6]. Additionally, the sleep-wake cycle in AD patients is frequently disrupted by nocturnal awakenings and reduced daytime wakefulness following naps [7-9].
The alterations in sleep architecture are believed to worsen the pathology of cerebral AD. Specifically, during deep sleep, there is a reduced clearance of Ab-amyloid in the brain, leading to enhanced deposition and ultimately contributing to the worsening of cognitive symptoms [10]. However, accumulating evidence suggests that there is a bidirectional relationship between sleep and AD. It is not just a symptom of AD, but poor-quality sleep, insufficient sleep, and excessive daytime napping are also proposed to increase the risk of developing AD in the future [11]. A metaanalysis conducted in 2017 supports this notion, indicating that individuals with sleep disturbances have a 1.55 times higher risk of developing AD compared to those without sleep disturbances. Additionally, poor sleep accounts for up to 15% of the population attributable risk for AD [12]. It is important to note that this estimate may underestimate the true extent of the problem, as many of the studies included in the analysis relied on self-reported sleep measures rather than objective assessments.
Sleep is regulated by two processes: the homeostatic process and the circadian process. These processes control various aspects of sleep behavior and associated variables. The protein Sirtuin 1 (Sirt 1) is involved in both the circadian rhythm and the homeostatic process of sleep. It is associated with wake-sleep neurotransmitters and somnogens, further highlighting its role in regulating sleep [13,14]. Accordingly, the role of Sirt 1 in cell survival is of great significance, as it is believed to provide protection against the deposition of Ab-amyloid and the development of AD-related tau pathology [15,16]. In this review, we aim to explore the potential connections between Sirt 1, its encoding gene SIRT1, sleep regulation, and AD. Additionally, we will discuss various activators of Sirt 1 in the context of AD prevention, and propose recommendations for future research that may contribute to a deeper understanding of the intricate and multifaceted relationship between Sirt 1/SIRT1, sleep, and the etiology of AD (Figure 1).
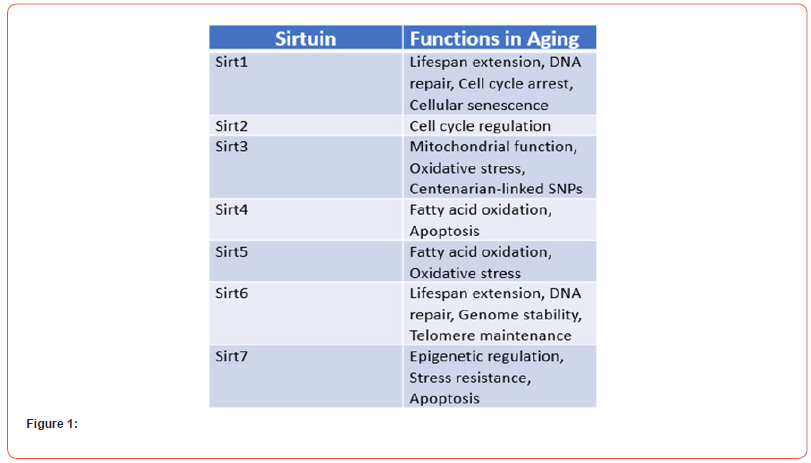
Sirtuins, also referred to as Silent information regulator 2 (Sir2) proteins, were initially discovered in yeast (Saccharomyces cerevisiae). They belong to a conserved family of nicotinamide adenine dinucleotide (NAD)-dependent protein deacetylases and are classified as class III histone deacetylases (HDACs). Sirtuins play a crucial role in the removal of acetyl groups from both histones and non-histone proteins, including transcription factors and enzymes. Among the seven sirtuins found in humans and other mammals, Sirt 1 is the most extensively studied and will be the main focus of this review [17]. The SIRT1 gene, responsible for encoding Sirt 1, is located on chromosome 10 (Chr10q21.3) in humans. These enzymes, known for their potential anti-aging effects, tend to decrease in levels as individuals age [18]. Consequently, they are considered promising candidates for the investigation of age-related diseases such as Alzheimer’s disease (AD) [18,19]. Sirt 1 has been associated with various biological processes in mammals, including aging, calorie restriction, metabolism, cancer, stress responses, chromosomal stability, cell differentiation, and the regulation of the circadian clock [20,21]. The exact mechanisms underlying the decline of Sirt 1 with age remain unknown. However, its reduction has been observed in both human and animal models during midlife, a period when age-related changes in sleep disorders and wakefulness commonly occur [22,23].
Methods and Materials
The following databases were searched for this review article: To find the most significant comparative research on the relationship between sleep and brain health, its therapeutic options, search engines like Google Scholar, PubMed, and Directory Open Access Journal databases. Keywords like sleep, brain health, slow wave sleep, NREM sleep, amyloid precursor protein, Sirtuin, Alzheimer’s disease, sleep disorders, retinoic acid, amyloid production, and drugs are also used. After assessing the quality and strength of the findings, meta-analyses, systematic reviews, large epidemiological studies, and randomized control trials were used as the main sources of information where they were available (Figure 2).
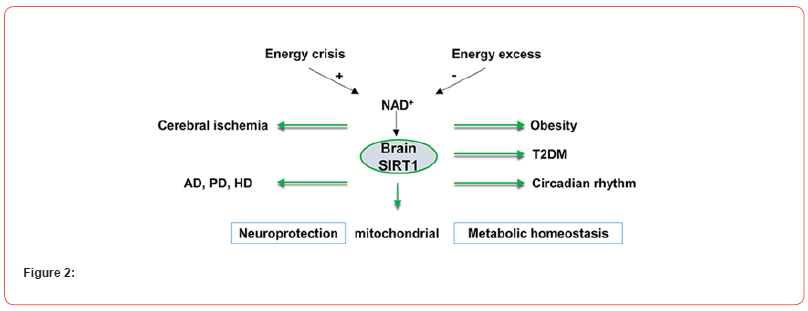
Results
Alzheimer
Regarding Alzheimer’s disease, Sirt 1 has been proposed to have a potential protective effect by modulating the acetylation homeostasis of proteins and enzymes associated with the disease [19,24]. One of the hallmarks of AD is the accumulation of Abamyloid peptides in the brain, resulting from the sequential cleavage of the amyloid precursor protein (APP) by the enzyme beta-secretase. Conversely, activation of alpha-secretase suppresses Ab-amyloid production [16]. Sirt 1, deacetylation of transcriptional factors related to alpha-secretase and beta-secretase enzymes, may reduce the burden of Ab-amyloid in the brain [15,16]. For instance, Sirt 1 directly promotes the transcription of the gene encoding alpha-secretase. This is achieved by removing acetyl groups from the retinoic acid receptor beta, a key regulator of alpha-secretase transcription. Additionally, the induction of alpha-secretase by Sirt 1 activates the Notch pathway, which plays a role in the repair of damaged neurons [15].
Sirt 1 has been found to play a role in the deacetylation of tau, which leads to the ubiquitination of hyperphosphorylated tau. This process facilitates the proteasomal degradation of tau and prevents the formation of tau tangles, a hallmark of Alzheimer’s disease (AD) [25]. Studies have shown that Sirt 1 is expressed in neurons of the hippocampus, a brain region critical for memory and learning functions that are impaired in AD [21,26]. In AD, there is a reduction in Sirt 1 expression in the brain, which correlates with the accumulation of AD pathology and disease progression [27,28]. Additionally, cognitive disorders have been observed in SIRT1 knockout mice [20], and dysregulation of the Sirt 1 pathway has been implicated as a critical mediator of AD pathogenesis in animal models [29]. Importantly, animal studies have demonstrated that SIRT1 gene expression in the hippocampus and other brain regions can be increased through interventions such as dietary restriction or physical exercise, suggesting potential approaches for enhancing Sirt 1-mediated neuroprotection [18,30].
Sirt 1 and Sleep
The relationship between Sirt 1 and sleep will be discussed in more detail later. Sleep in mammals is categorized into different stages based on cortical electroencephalography (EEG) activity, including REM sleep and non-REM (NREM) sleep, which consists of three sub-stages: N1, N2, and N3 (also known as slow-wave sleep). According to conventional sleep models, wake-promoting neurons (WPNs) and sleep-promoting neurons (SPNs) create a “switch” system in which they compete for network dominance. In the human brain, WPNs and SPNs are primarily located in the brainstem and diencephalon, accounting for less than 1% of neurons. These neurons are associated with different neurotransmitters and have opposing modulatory effects [31].
SPNs are believed to play a role in controlling sleep by inhibiting wake-promoting centers, such as GABAergic neurons in the ventrolateral preoptic nuclei and melanin-concentrating hormone (MCH)-producing neurons in the diencephalon [33,34]. This suggests that the regulation of sleep involves multiple molecular mechanisms and genes, rather than a singular one. Sleep physiology can be examined from two different perspectives: 1) the timing of sleep, which is regulated by the circadian process (process C) in the brain and is independent of sleep itself, and 2) the duration of sleep, which is influenced by the homeostatic, sleep-dependent process (process S) [35,36]. There is a potential interaction between process C and process S in the regulation of sleep [37]. In humans, process C is demonstrated by REM sleep and exhibits a circadian rhythm that closely correlates with body temperature. These processes are minimally affected by sleep and wakefulness within the past 24 hours [38]. In contrast, external factors such as prior wake or sleep can impact process S, which determines N3, and slow-wave activity in EEG [35].
The Circadian System
The circadian system plays a crucial role in regulating sleep timing. Circadian clock regulators are divided into positive and negative categories. Brain and Muscle ARNT-Like 1 (BMAL1; also known as Aryl hydrocarbon receptor nuclear translocator-like protein 1, ARNTL) and Circadian Locomotor Output Cycles Kaput (CLOCK, and its paralogue Neuronal Per-Arnt-Sim domain protein 2, NPAS2) are positive regulators in mammals. These ‘master genes’ drive rhythmic gene expression and control biological functions under circadian regulation. BMAL1: CLOCK protein heterodimers initiate the transcription of target genes, including those encoding periods (Pers) and cryptochromes (Crys), which are negative circadian clock regulators. The resulting proteins form dimers that inhibit further transcription of BMAL1 and CLOCK (Figure 3). This negative transcriptional feedback loop allows the cycle to repeat through a low level of transcriptional activity, thereby creating a 24-hour rhythm in mammals [39]. CLOCK protein exhibits histone acetyltransferase (HAT) activity. Studies on animals have demonstrated that Sirt 1, with its deacetylase function, counteracts the HAT activity of CLOCK, subsequently influencing the expression of Cry1, Per1, and Per2 in mice [40-42]. Deletion of Sirt1 in mouse models results in disruption of circadian rhythm and an inability to adapt to a new light-dark cycle. The decrease in both SIRT1 expression and NAD+ levels with aging could be a potential mechanism for the weakening of circadian control in older adults [13].
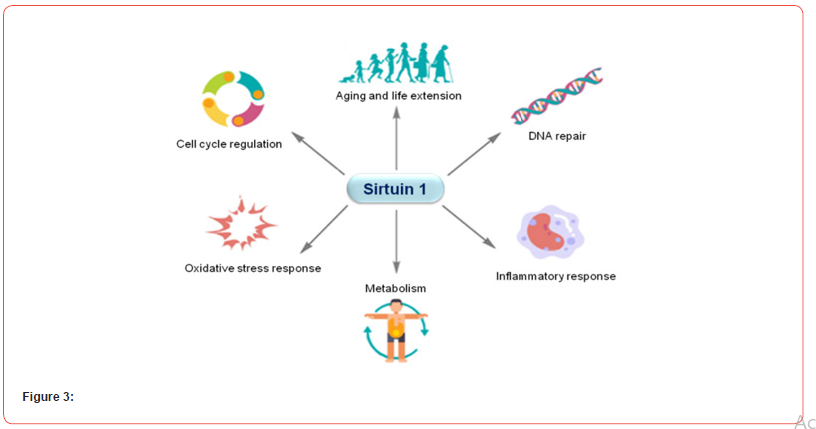
The Circadian System
The circadian system plays a crucial role in regulating the timing of sleep, while the homeostatic process is responsible for controlling the duration of sleep. The homeostatic sleep drive, which is the pressure to sleep, increases as the time spent awake increases, and decreases during sleep, returning to a ‘baseline’ level after a night of good quality sleep. In cases of sleep deprivation, lost sleep can be compensated for by increasing the amount of sleep. Conversely, excessive sleep leads to a decrease in the inclination to sleep. The homeostatic process is considered a fundamental regulatory mechanism for sleep. Delta waves, with a frequency of 0.5-4 Hz recorded through EEG, are typically linked to N3 (slow wave) sleep, defining deep sleep, and are controlled by the homeostatic process [43].
Animal studies have suggested that Sirt 1 may play a role in maintaining delta waves during NREM sleep [23,44]. Consistent with this idea, research on humans has shown that delta power in EEG decreases with age, which is in line with the reduced levels of Sirt 1 in aging individuals. It is worth noting that delta power is not only associated with sleep quality but also with longevity and metabolic complications [45,46]. Therefore, it is plausible to suggest that Sirt 1 contributes to sleep homeostasis, although the exact mechanism behind this relationship requires further investigation.
Satoh et al. conducted a study on transgenic mice that overexpressed Sirt 1 specifically in the brain (BRASTO mice) and found that these aged mice had higher delta power during NREM sleep compared to control mice. Interestingly, there was no difference in delta power during wakefulness, indicating that the aged BRASTO mice experienced higher quality or deeper sleep [44]. Similarly, another study on mice with knockdown of SIRT1 in specific brain regions (dorsomedial and lateral hypothalamic nuclei) showed a decrease in sleep quality [23]. A recent study in mice proposed that Sirt 1 may mediate sleep quality through the Sirt 1/Nk2 homeobox 1 (Nkx2-1)/orexin type 2 receptor (Ox2r) pathway, which is a major pathway involved in maintaining delta power during NREM sleep [44].
Sleep Disturbances
Sleep disturbances are well-established in individuals with dementia due to Alzheimer’s disease (AD) [47]. Importantly, evidence suggests that these sleep disturbances not only coexist with AD but also increase the risk of cognitive decline and dementia [11,48,49]. This is believed to occur through the modulation of neurobiological changes in the brain, including increased atrophy, accumulation of brain Ab-amyloid and tau pathology (hallmarks of AD), as well as reduced brain glucose metabolism [50-54]. Recent research indicates the significance of sleep quality in relation to the functioning of the glymphatic system in the brain [10]. Studies have shown that an increase in delta power and a decrease in heart rate during sleep are associated with improved glymphatic flow [55]. Similar to the lymphatic system in other organs, the glymphatic system acts as a “housekeeping” system for the brain [56], removing waste products such as tau oligomers and Ab-amyloid protein, which are linked to Alzheimer’s disease and cognitive function [57]. Additionally, findings from studies conducted on mice suggest that an increase in delta power during recovery sleep specifically enhances cognitive performance that relies on the prefrontal brain regions [58].
Changes in circadian rhythms have been observed in both healthy aging and age-related diseases like Alzheimer’s disease. A recent review has summarized evidence of age-related alterations in various aspects of the circadian system, including: 1) reduced expression of circadian-related genes like CLOCK and BMAL1, 2) changes in structures responsible for light transmission and processing, such as the pupil and retina, and 3) decreased amplitude of rhythmic behaviors, disturbances in circadian timing, and an increased prevalence of sleep disorders [59]. Even in healthy older adults without clinical symptoms of sleep disorders, the aging process is associated with a decline in sleep quality and quantity, reduced sleep depth and intensity, compromised sleep integrity, and a higher frequency of daytime napping [60].
Accumulating evidence suggests that circadian rhythm disruption in age-related diseases, such as Alzheimer’s disease, is even more pronounced. This disruption has been proposed as a biomarker for the presence and severity of Alzheimer’s pathology [61]. Indeed, several key processes implicated in the development of Alzheimer’s disease are believed to follow a circadian rhythm, including cerebral blood flow, glymphatic system function, Abamyloid clearance, melatonin production, and metabolism [61].
Orexin
Orexin, a substance that affects both WPNs and SPNs in the brain, plays a crucial role in maintaining stable sleep and wake cycles [66]. However, its main function seems to be in generating a signal that promotes wakefulness. Research suggests that orexin and its receptors also contribute to the development and progression of neurodegenerative diseases like Alzheimer’s disease (AD) [67]. There have been reports of dysregulation in the orexin system in the presence of AD pathology, leading to changes in sleep patterns [68,69]. In individuals with mild cognitive impairment, which often precedes AD, increased levels of orexin in the cerebrospinal fluid have been associated with impaired REM sleep [70,71]. However, the exact mechanism by which alterations in the orexin system worsen sleep in AD still needs to be clarified. It is worth noting that orexin also plays a critical role in regulating stress. Since stress and stress hormones are linked to both AD and reduced sleep quality, this additional function of orexin should be taken into account when considering its relationship with sleep and AD.
The gene encoding the orexin type 2 receptor (OX2R) is a key target of Sirt 1 in the hypothalamus. Sirt 1 has been observed to increase the expression of OX2R, particularly in the dorso-medial and lateral regions of the hypothalamus. The OX2R pathway, which also involves the orexin type 1 receptor, plays a crucial role in arousal, physical activity motivation, and metabolism. Studies have shown that transgenic mice with increased Sirt 1 levels in the brain exhibit enhanced longevity and delayed aging due to the dosedependent upregulation of OX2R. These findings suggest a potential mechanism by which Sirt 1 influences sleep and Alzheimer’s disease through the orexin system [72].
Acetylcholine (ACh)
Acetylcholine (ACh) is an essential component of the arousal system, playing a critical role in wakefulness and REM sleep. However, direct analysis of ACh has been challenging, leading some to believe that ACh does not directly regulate sleep, but rather sleep stages regulate ACh levels. In Alzheimer’s disease, the cholinergic nuclei in the basal forebrain degenerate, resulting in decreased activity of choline acetyltransferase and acetylcholinesterase, which contributes to cholinergic dysregulation in AD patients. The decline in cholinergic tone may be responsible for cognitive and arousal impairments [73].
Sirt 1 has been found to influence the expression of ACh receptors and choline levels in the brain, suggesting another potential mechanism through which Sirt 1 may impact sleep and Alzheimer’s disease. Cytidine-50-diphosphate-choline (CDPcholine) is a naturally occurring compound that serves as a source of choline in metabolic pathways for ACh biosynthesis. CDP-choline has neuroprotective properties and has been shown to have significant positive effects on memory and behavior [5,14,74]. A recent investigation demonstrated that CDP-choline has the ability to enhance the expression of Sirt1 protein in the rat brain, providing neuroprotection [75]. Additionally, it has been proposed that alpha 7 nicotinic acetylcholine (ACh) receptors can enhance the activity of Sirt1 by increasing the levels of its cofactor NAD+ within the cells [76]. Furthermore, the anti-aging properties associated with these receptors seem to be mediated by Sirt1 [76]. Notably, in an animal model of Alzheimer’s disease (AD), treatment with an agonist of the alpha 7 nicotinic ACh receptor resulted in neuroprotective effects and improved learning and memory abilities [77]. It is plausible to suggest that these beneficial effects were mediated by Sirt1.
The role of monoaminergic neurotransmitter systems in the sleep-wake cycle has been extensively studied [5,31,32]. These systems are most active during wakefulness, exhibit reduced activity during non-rapid eye movement (NREM) sleep, cease functioning before and after rapid eye movement (REM) sleep, and resume firing before the onset of wakefulness [78]. Impairment of the monoaminergic system is commonly observed in AD. Due to the relatively low number of monoaminergic fibers in the brain and the fact that these neurons have long, unmyelinated axons, they are more susceptible to neurological abnormalities associated with AD pathology. Specifically, monoaminergic neurons project into the hippocampus and cortical regions that are significantly affected by the accumulation of hyperphosphorylated tau and Ab-amyloid [79].
Sirt1 is expressed in monoaminergic neurons, where its presence is crucial for normal wakefulness and the proper functioning of wake-active neurons. Studies involving transgenic animals and the conditional loss of brain Sirt1 in adult mice have shown that the absence of Sirt1 leads to significant disturbances in wakefulness and a reduction in wake time, without affecting sleep consolidation [23]. Furthermore, this research also revealed an agerelated decline in Sirt1 levels specifically in wake-active neurons, excluding serotoninergic wake-active neurons [23].
Another study conducted on mice found that SIRT1, a protein, can regulate the levels of monoamines by deacetylating NHLH2, a transcription factor for the gene MAO-A. MAO-A encodes an enzyme called monoamine oxidase A, which is involved in the breakdown of monoamine neurotransmitters [80]. Additionally, research conducted in a laboratory setting demonstrated that overexpression of a specific microRNA called miR-142 led to a decrease in neuronal expression and enzymatic activity of MAO-A through the downregulation of SIRT1 [81]. Abnormal expression of microRNAs has been linked to the development of various neurodegenerative disorders, potentially through the influence of SIRT1 on neurotransmission. Therefore, the modulation of monoaminergic neurotransmitter systems represents another possible mechanism through which SIRT1 can impact sleep and Alzheimer’s disease.
MCH
Melanin-concentrating hormone (MCH), a neuropeptide, plays a role in reducing locomotor activity, conserving energy, and promoting sleep when there is a surplus of energy [82]. It is also believed to be involved in negative energy balance by reducing activity and REM sleep [83]. Depletion of MCH, either through genetic knockout or the use of an antagonist, is thought to decrease both NREM and REM sleep, increase sleep fragmentation, and heighten alertness [84]. Animal studies have indicated that increased levels of MCH can lead to improved memory, learning, and performance [85].
There is emerging evidence indicating that the function of MCH (melanin-concentrating hormone) is disrupted in Alzheimer’s disease (AD), and this disruption is associated with AD-related characteristics such as the presence of neurofibrillary tangles composed of hyperphosphorylated tau proteins. MCH receptors are widely distributed in the hippocampus and cortex, which are regions particularly susceptible to AD-related neuropathology and neurodegeneration. Consequently, the disturbed function of MCH in AD and its potential effects on learning and memory performance may be attributed to a decrease in MCH receptors.
A recent study has provided evidence that Sirtuin 1 (Sirt 1), a protein involved in various cellular processes, including sleep regulation, plays a role in the regulation of MCH in neurons that express pro-opiomelanocortin (POMC), which promote sleep. Therefore, inhibiting Sirt 1 in these regions could potentially impair MCH functions. Additionally, the Sirt 1/Forkhead Box O1 (FoxO1)/POMC signaling pathway has been proposed as a potential regulatory mechanism for MCH activity. This pathway is believed to contribute to the regulation of energy balance, food intake, and the sleep-wake cycle. Taken together, these findings suggest that MCH could be another target through which Sirt 1 may influence sleep and AD [86,87].
Adenosine
Adenosine, along with its receptors, plays a crucial role in regulating both circadian rhythm and the sleep drive. Adenosine itself possesses sleep-inducing properties and is considered a “sleep substance” that modulates the sleep-wake cycle through its A1 and A2A receptor subtypes, which are the most prevalent receptor subtypes in the mammalian brain. Studies have demonstrated that a decrease in its derivative adenosine triphosphate (ATP) and an increase in extracellular adenosine levels are positively associated with sleep patterns [88,89]. Activation of A1 receptors enhances slow-wave activity [90], which is the primary indicator of homeostatic sleep regulation. Additionally, caffeine has been suggested to influence the sleep-wake pattern through A2A receptors [91]. The signaling molecule cyclic AMP, derived from adenosine, is itself a component of the circadian clock and indirectly triggers the transcription of numerous circadian genes while also influencing cell cycle timing. AMP kinase, a cellular energy sensor that relies on AMP, can phosphorylate multiple clock proteins, including Sirt 1, thereby upregulating this enzyme and affecting downstream pathways. As mentioned earlier, the function of Sirt 1 is also influenced by NAD+ cofactor levels, which are regulated by both circadian and metabolic processes [92]. The neuromodulatory role of adenosine and its receptors has been observed in various neurodegenerative conditions, including Alzheimer’s disease (AD) [93]. Epilepsy is reported in 10% to 22% of AD patients, often occurring during the early stages of the disease or even before the formation of cerebral Ab-amyloid plaques [94]. It is believed that global DNA hypermethylation is associated with chronic epilepsy [95], and disruption of adenosine homeostasis is implicated in this mechanism. Consequently, therapeutic administration of adenosine may improve DNA methylation profiles and potentially alleviate the progression of epilepsy [94].
A recent investigation revealed that Sirt 1 in the brain plays a crucial role in managing epilepsy, while adenosine enhances epigenetic modifications, neuron survival, and synaptic plasticity [93]. However, the impact of adenosine impairment on AD pathophysiology is intricate. In the short term, increased adenosine levels could potentially have therapeutic benefits by suppressing methyltransferase and subsequently reducing DNA methylation changes, which are commonly observed in the brains of AD patients [96]. Additionally, elevated adenosine levels and the resulting increased A1 receptor activity improve the hyperexcitability and excitotoxicity network in AD parenchyma. Conversely, heightened A2 receptor activity due to increased adenosine levels leads to memory deficits and AD pathology. Overall, these findings suggest that the positive effects of elevated adenosine levels are likely dependent on the specific receptor subtype [96].
Melatonin
Melatonin, a metabolite of the amino acid tryptophan, is produced in the pineal gland. In humans, melatonin contributes to various physiological processes, including the regulation of circadian rhythm and sleep physiology. Following two hours of endogenous melatonin secretion at night, sleep propensity significantly increases [97]. In diurnal species, melatonin reduces the wake-promoting signal of the circadian clock, thereby promoting sleep [98]. However, nocturnal melatonin secretion is disrupted with advancing age and in neurodegenerative disorders such as AD, leading to abnormal sleep patterns [99]. Melatonin secretion decreases in individuals with mild cognitive impairment, which is often the earliest manifestation of AD neuropathology [100,101] and continues to decline as the disease progresses [100]. A recent study indicated that mild cognitive impairment patients with alterations in melatonin production experience disturbances in the circadian clock, resulting in increased wakefulness at night and prolonged REM latency [102].
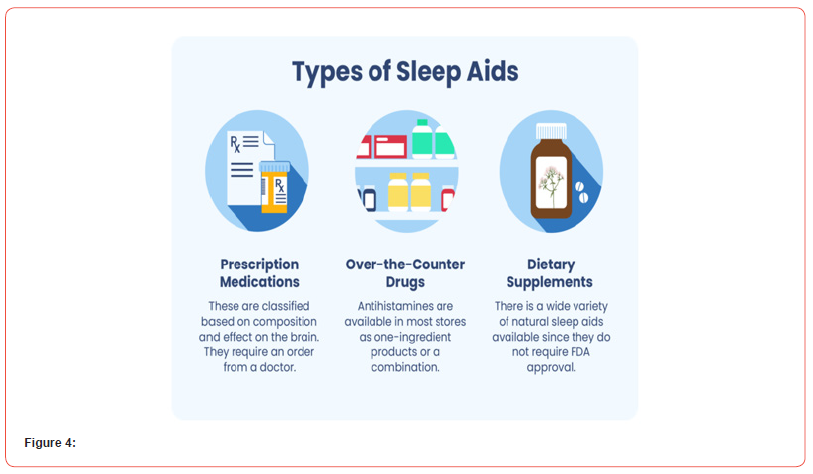
One possible explanation for these effects could be the excessive regulation of monoamine oxidase in AD, which leads to a decrease in serotonin, the precursor of melatonin [103]. Studies conducted on animals and in vitro also indicate that administering melatonin can improve AD pathology by potentially reducing the production of Ab-amyloid through increased secretase activity and decreased b-and c-secretases [104-106]. Additionally, melatonin has been found to up-regulate ADAM10 (A Disintegrin and Metalloproteinase 10) in vitro by activating the SIRT1 pathway [104]. ADAM10 is the primary a-secretase in neurons and is responsible for cleaving APP in a way that suppresses Ab-amyloid production. These in vitro findings are supported by an animal study where longterm melatonin administration to aged mice resulted in positive changes to secretase activity in the hippocampus, accompanied by a decrease in phosphorylated NF-jB and an increase in Sirt 1 [106]. The mice also showed improved spatial memory following melatonin treatment. Based on these findings, the authors suggest that dietary supplementation to counteract age-related melatonin loss could be a potential therapeutic approach for preventing and managing AD [106]. The effects of melatonin treatment have also been studied in rats subjected to total sleep deprivation [107]. After total sleep deprivation, the rats exhibited significant impairment in spatial memory and reduced Sirt 1 levels in the hippocampus. However, when the sleep-deprived rats were given melatonin doses of 5, 25, 50, or 100 mg/kg/day, the expression of Sirt 1 in the hippocampus was preserved. These neurobiological benefits of melatonin treatment were accompanied by improved performance in behavioral tests. As a result, the authors propose melatonin as a potential therapeutic strategy for preventing memory deficits caused by total sleep deprivation and suggest that Sirt 1 may play a role in mediating these beneficial effects [107]. Numerous studies have also emphasized the significant involvement of Sirt 1 in regulating melatonin function to enhance insulin resistance, aging, and anti-inflammatory properties; [108-110] all of which have implications for Alzheimer’s disease (AD) risk and development. The cumulative findings in this section propose melatonin as an additional target by which Sirt 1 could potentially influence sleep and AD (Figure 4).
Retinal
The function of the retinal in mammals involves the localization of the functional photopigment melanopsin within intrinsically photosensitive retinal ganglion cells (ipRGCs), which plays a role in non-visual photoreceptive functions. The functions of melanopsin illustrate that light not only communicates with the circadian clock but also interacts intricately with various neurological and pathological processes [111,112]. The response to light by the body is crucial for the regulation of rhythmic physiological functions, such as hormonal cycles, and the expression of negative circadian clock regulator genes Per and Cry. Consequently, ipRGCs play a significant role in the adjustment of the circadian clock. The depletion of ipRGCs, whether acquired or inherited in neurodegenerative diseases, results in the impairment of dopaminergic neurons in the retina. Similarly, disruptions in light detection or transmission to the retina due to aging or conditions like cataracts, lead to the dysregulation of circadian synchrony and subsequent impairment of various physiological processes, including sleep. In line with these discoveries, the decrease in melanopsin levels seems to be associated with insomnia, depression, and cognitive decline [112,113].
Blue light at 460 nm has been suggested to enhance cognitive functions both during the day and at night [114]. However, the disruption of the circadian clock may impact the effects of exposure to the blue light spectrum, particularly in older individuals leading to decreased melatonin levels and reduced alertness [115,116]. Studies have shown retinal thinning and vascular disturbances in the retina of individuals with Alzheimer’s disease (AD). Additionally, AD-related markers such as Ab-amyloid plaques, hyperphosphorylated tau, and neurodegeneration have been identified in the retinas of individuals with early signs of AD. Recent research indicates that retinal scans could potentially aid in the early diagnosis of AD [117-119]. Sirt 1, found in various parts of the eye, plays a crucial role in maintaining retinal health and function. In mice lacking SIRT1, abnormalities in retinal cell layers and increased apoptosis of retinal progenitor cells have been observed. Sirt 1 influences aging, inflammation, oxidative stress, angiogenesis, and neuroprotection in the retina and ocular systems. The expression of SIRT1 in retinal tissue is influenced by light, with levels increasing at night, suggesting a tissue-specific regulation of SIRT1 in the eye [120-125].
Discussion
The presence and severity of Alzheimer’s retinopathy are likely to have a negative impact on the transmission of light to the retina, which in turn affects various physiological functions such as sleep and the expression of SIRT1 [126]. Activators of Sirt 1, including dietary factors and other modifying strategies, have been proposed to counteract these effects. Several approaches, such as intermittent fasting, calorie restriction, and exercise combined with calorie restriction, have been shown to increase the levels of Sirt 1 mRNA in human muscle tissue. Animal studies have also demonstrated that calorie restriction can elevate Sirt 1 levels in the brain, particularly in the vulnerable hypothalamus region affected by AD neuropathology [127,128]. Additionally, compounds like Rhein derived from rhubarb and DOPET found in olive oil, grape juice, and wine have shown potential therapeutic effects in animal models of AD by activating the Sirt 1 pathway and improving mitochondrial biogenesis. However, further research is needed to determine the efficacy of these treatments in humans [129].
The increase in SIRT1 activity observed in this study was accompanied by enhanced clearance of neurotoxic Abamyloid through a-secretase-mediated mechanisms, resulting in neuroprotection. Resveratrol, a polyphenol found in grapes and grape products like red wine, has been well-established as an activator of Sirt 1 both in laboratory settings and in living organisms [130-133]. Furthermore, studies conducted on patients with Alzheimer’s disease (AD) have shown that high doses of resveratrol can reduce AD biomarker levels in cerebrospinal fluid, provide neuroprotection in brain regions affected by early AD, and slow cognitive decline while maintaining function in individuals with mild to moderate AD. It is important to note, however, that the beneficial effects of resveratrol are not solely dependent on Sirt 1 activation, as this compound has been found to modulate various intracellular signaling pathways. The impact of Sirt 1/SIRT1- activating strategies, such as those mentioned above, on AD risk and sleep-related factors, remains to be determined, as does their potential therapeutic role in AD treatment.
In terms of future research directions, accumulating evidence from both animal and human studies suggests a complex and multifaceted relationship between Sirt 1/SIRT1, sleep, and the development of AD. However, further research is needed to fully understand this relationship. One recommendation for future studies is to simultaneously measure Sirt 1 levels and sleep parameters in well-characterized longitudinal cohorts of aging individuals and those with AD. By assessing Sirt 1 levels in plasma, serum, or cerebrospinal fluid, along with sleep efficiency, duration, and stages, across the continuum of AD progression (from preclinical to prodromal and dementia stages), we can gain a better understanding of the relationship between Sirt 1, sleep, and the progression of AD.
Furthermore, by including individuals who maintain their cognitive abilities over time (both with and without AD biomarkers), the study can also consider the impact of healthy aging compared to pathological aging. This analysis is crucial for understanding how age influences the relationship between Sirt 1/SIRT1, sleep, and AD.
Age is the greatest risk factor for AD, and both sleep patterns and Sirt 1 are associated with the aging process. Once the relationship between Sirt 1/SIRT1, sleep, and AD etiology is well understood, the next logical step would be to investigate the effects of Sirt 1 activators (such as resveratrol, intermittent fasting, and calorie restriction) on sleep patterns and AD biomarkers simultaneously. This research could provide insights into how these approaches could potentially be used to delay or prevent the onset of ADrelated dementia.
Conclusion
A growing body of evidence from animal and human studies supports a significant and complex relationship between Sirt 1/ SIRT1, sleep, and AD. Various hypotheses have been proposed to explain the critical role of Sirt 1/SIRT1 in the mechanisms and neurotransmitters related to wake-promoting neurons (WPNs) and sleep-promoting neurons (SPNs). However, there is a lack of studies investigating the interaction between sleep and Sirt 1/SIRT1, a key component of the circadian clock, in relation to AD pathology. This review has explored the potential association between Sirt 1/ SIRT1, sleep, and AD etiology. Considering that sleep is a modifiable risk factor for AD and recent studies suggest that Sirt 1/SIRT1 activation can be influenced by lifestyle and dietary approaches, further research is needed to explore its potential as a target for AD prevention and treatment.
Acknowledgement
None.
Conflicts of Interest
No Conflict of Interest.
References
- Daley M, Morin CM, LeBlanc M, Grégoire J-P, Savard J, et al. (2009) Insomnia and its relationship to health-care utilization, work absenteeism, productivity and accidents. Sleep Med 10 (4): 427-438.
- Musiek ES, Holtzman DM (2016) Mechanisms linking circadian clocks, sleep, and neurodegeneration. Science 354(6315): 1004-1008.
- Ancoli-Israel S (2005) Sleep and aging: prevalence of disturbed sleep and treatment considerations in older adults. J Clin Psychiatry 66 Suppl 9: 24-30.
- Cooke JR, Ancoli-Israel S (2006) Sleep and its disorders in older adults. Psychiatric Clinics 29(4): 1077-1093.
- Van Erum J, Van Dam D, De Deyn PP (2019) Alzheimer’s disease: neurotransmitters of the sleep-wake cycle. Neuroscience & Biobehavioral Reviews 105: 72-80.
- PN Prinz, ER Peskind, PP Vitaliano, MA Raskind, C Eisdorfer, et al. (1982) Changes in the sleep and waking EEGs of nondemented and demented elderly subjects. J Am Geriatr Soc 30(2): 86-92.
- Bliwise DL, Watts RL, Watts N, Rye DB, Irbe D, et al. (1995) Disruptive nocturnal behavior in Parkinson’s disease and Alzheimer’s disease. J Geriatr Psychiatry Neurol 8(2): 107-110.
- Huang YL, Liu RY, Wang QS, Van Someren EJ, Xu H, et al. (2002) Age-associated difference in circadian sleep–wake and rest–activity rhythms. Physiol Behav 76(4-5): 597-603.
- Prinz PN, Vitiello MV, Raskind MA, Thorpy MJ (1990) sleep disorders and aging. N Engl J Med 323(8): 520-526.
- Lulu Xie, Hongyi Kang, Qiwu Xu, Michael J Chen, Yonghong Liao, et al. (2013) Sleep drives metabolite clearance from the adult brain. Science 342(6156): 373-377.
- Peng Li, Lei Gao, Lei Yu, Xi Zheng, Ma Cherrysse Ulsa, et al. (2023) Daytime napping and Alzheimer’s dementia: A potential bidirectional relationship. Alzheimers Dement 19(1): 158-168.
- Omonigho M Bubu, Michael Brannick, James Mortimer, Ogie Umasabor-Bubu, Yuri V Sebastião, et al. (2017) Sleep, Cognitive impairment, and Alzheimer’s disease: A Systematic Review and Meta-Analysis. Sleep: 40(1).
- Chang HC, Guarente L (2013) SIRT1 mediates central circadian control in the SCN by a mechanism that decays with aging. Cell 153 (7):1448-1460.
- Huang PS, Son JH, Abbott L, Winzer-Serhan U (2011) Regulated expression of neuronal SIRT1 and related genes by aging and neuronal b2-containing nicotinic cholinergic receptors. Neuroscience 196: 189-202.
- Saftig P, Lichtenthaler SF (2015) The alpha secretase ADAM10: A metalloprotease with multiple functions in the brain. Prog Neurobiol 135: 1-20.
- Hye Rin Lee, Hwa Kyoung Shin, So Youn Park, Hye Young Kim, Won Suk Lee, et al. (2014) Cilostazol suppresses b-amyloid production by activating Disintegrin and metalloproteinase 10 via the upregulation of SIRT1-coupled retinoic acid receptor-b.J Neurosci Res 92(11): 1581-1590.
- Finkel T, Deng CX, Mostoslavsky R (2009) Recent progress in the biology and physiology of sirtuins. Nature 460(7255): 587-591.
- Quintas A, de Solís AJ, Díez-Guerra FJ, Carrascosa JM, Bogónez E, et al. (2012) Age-associated decrease of SIRT1 expression in rat hippocampus: prevention by late onset caloric restriction. Exp Gerontol 47(2): 198-201.
- Donmez G (2012) The neurobiology of sirtuins and their role in neurodegeneration. Trends Pharmacol Sci 33(9): 494-501.
- Shaday M, Ying L, Maggie MHC, Edoardo P, Huanying G, et al. (2010) SIRT1 is essential for normal cognitive function and synaptic plasticity. J Neurosci 30(29): 9695-9707.
- Bordone L, Guarente L (2005) Calorie restriction, SIRT1 and metabolism: understanding longevity. Nat Rev Mol Cell Biol 6(4): 298-305.
- Colas D, Cespuglio R, Sarda N (2005) Sleep wake profile and EEG spectral power in young or old senescence accelerated mice. Neurobiol Aging 26(2): 265-273.
- Panossian L, Fenik P, Zhu Y, Zhan G, McBurney MW, et al. (2011) SIRT1 regulation of wakefulness and senescence-like phenotype in wake neurons. J Neurosci 31(11): 4025-4036.
- Herskovits AZ, Guarente L (2014) SIRT1 in neurodevelopment and brain senescence. Neuron 81(3): 471-483.
- Sang-Won Min, Seo-Hyun Cho, Yungui Zhou, Sebastian Schroeder, Vahram Haroutunian, et al. (2010) Acetylation of tau inhibits its degradation and contributes to tauopathy. Neuron 67(6): 953-966.
- Mu Y, Gage FH (2011) Adult hippocampal neurogenesis and its role in Alzheimer’s disease. Mol Neurodegener 6(1): 85.
- Carl Julien, Cyntia Tremblay, Vincent Emond, Meryem Lebbadi, Norman Salem, et al. (2009) Sirtuin 1 reduction parallels the accumulation of tau in Alzheimer disease. J Neuropathol Exp Neurol 68(1): 48-58.
- Lutz MI, Milenkovic I, Regelsberger G, Kovacs GG (2014) Distinct patterns of sirtuin expression during progression of Alzheimer’s disease. NeuroMol Med 16(2): 405-414.
- Ting Sun, Kaiyue Zhao, Mimin Liu, Zhongdi Cai, Li Zeng, et al. (2022) miR-30a-5p induces Abeta production via inhibiting the nonamyloidogenic pathway in Alzheimer’s disease. Pharmacol Res 178: 106153.
- Revilla S, Suñol C, García-Mesa Y, Giménez-Llort L, Sanfeliu C, et al. (2014) Physical exercise improves synaptic dysfunction and recovers the loss of survival factors in 3xTg-AD mouse brain. Neuropharmacology 81: 55-63.
- Oh J, Petersen C, Walsh CM, Bittencourt JC, Neylan TC, et al. (2019) The role of co-neurotransmitters in sleep and wake regulation. Mol Psychiatry 24(9): 1284-1295.
- Saper CB, Scammell TE, Lu J (2005) Hypothalamic regulation of sleep and circadian rhythms. Nature 437(7063): 1257-1263.
- Alam MA, Kumar S, McGinty D, Alam MN, Szymusiak R, et al. (2013) Neuronal activity in the preoptic hypothalamus during sleep deprivation and recovery sleep. J Neurophysiol 111(2): 287-299.
- T Gallopin, P Fort, E Eggermann, B Cauli, P H Luppi, et al. (2000) Identification of sleeppromoting neurons in vitro. Nature 404(6781): 992-995.
- Borbély AA (1982) A two-process model of sleep regulation. Hum neurobiol 1(3): 195-204.
- Dauvilliers Y, Maret S, Tafti M (2005) Genetics of normal and pathological sleep-in humans. Sleep Med Rev 9(2): 91-100.
- Tobler I, Achermann P (2007) Sleep homeostasis Scholarpedia 2(10): 2432.
- Czeisler CA, Zimmerman JC, Ronda JM, Moore-Ede MC, Weitzman ED, et al. (1980) Timing of REM sleep is coupled to the circadian rhythm of body temperature in man. Sleep 2(3): 329-346.
- Jun Hirayama, Saurabh Sahar, Benedetto Grimaldi, Teruya Tamaru, Ken Takamatsu, et al. (2007) CLOCK-mediated acetylation of BMAL1 controls circadian function. Nature 450 (7172): 1086-1090.
- Etchegaray JP, Lee C, Wade PA, Reppert SM (2003) Rhythmic histone acetylation underlies transcription in the mammalian circadian clock. Nature 421(6919): 177-182.
- Yasukazu Nakahata, Milota Kaluzova, Benedetto Grimaldi, Saurabh Sahar, Jun Hirayama, et al. (2008) The NAD+-dependent deacetylase SIRT1 modulates CLOCK-mediated chromatin remodeling and circadian control. Cell 134(2): 329-340.
- RH Wang, Tingrui Zhao, Kairong Cui, Gangqing Hu, Qiang Chen, et al. (2016) Negative reciprocal regulation between Sirt1 and Per2 modulates the circadian clock and aging. Sci Rep 6: 28633.
- Lehmann D, Ozaki H, Pal I (1987) EEG alpha map series: brain micro-states by space-oriented adaptive segmentation. Electroencephalogr Clin Neurophysiol 67(3): 271-88.
- Akiko Satoh, Cynthia S Brace, Nick Rensing, Paul Cliften, David F Wozniak, et al. (2013) Sirt1 extends life span and delays aging in mice through the regulation of Nk2 homeobox 1 in the DMH and LH. Cell Metab 18(3): 416-430.
- Landolt H, Dijk DJ, Achermann P, Borbely AA (1996) Effect of age on the sleep EEG: slow-wave activity and spindle frequency activity in young and middle-aged men. Brain Res 738(2): 205-212.
- Mary Amanda Dew, Carolyn C Hoch, Daniel J Buysse, Timothy H Monk, Amy E Begley, et al. (2003) Healthy older adults’ sleep predicts all-cause mortality at 4 to 19 years of follow-up. Psychosom Med 65(1): 63-73.
- Hatfield CF, Herbert J, van Someren EJ, Hodges JR, Hastings MH, et al. (2004) Disrupted daily activity/rest cycles in relation to daily cortisol rhythms of home-dwelling patients with early Alzheimer’s dementia. Brain 127(Pt 5): 1061-1074.
- Olivier Potvin, Dominique Lorrain, Hélène Forget, Micheline Dubé, Sébastien Grenier, et al. (2012) Sleep quality and 1-year incident cognitive impairment in community-dwelling older adults. Sleep 35(4): 491-499.
- Sterniczuk R, Theou O, Rusak B, Rockwood K (2013) Sleep disturbance is associated with incident dementia and mortality. Curr Alzheimer Res 10(7): 767-775.
- Sexton CE, Storsve AB, Walhovd KB, Johansen-Berg H, Fjell AM (2014) Poor sleep quality is associated with increased cortical atrophy in community-dwelling adults. Neurology 83(11): 967-973.
- Lo JC, Loh KK, Zheng H, Sim SK, Chee MW (2014) Sleep duration and agerelated changes in brain structure and cognitive performance. Sleep 37(7): 1171–1178.
- Belinda MB, Stephanie RR, Victor LV, Michael W, Romola SB, et al. (2016) The Relationship between Sleep Quality and Brain Amyloid Burden. Sleep 39 (5): 1063-1068.
- Joseph RW, Bryce AM, Randolph FH, Anne M, Theresa MH, et al. (2019) Sleep as a Potential Biomarker of Tau and beta-Amyloid Burden in the Human Brain. J Neurosci 39(32): 6315-6324.
- RS Osorio, E Pirraglia, T Gumb, J Mantua, I Ayappa, et al. (2014) Imaging and cerebrospinal fluid biomarkers in the search for Alzheimer’s disease mechanisms. Neurodegener Dis 13(2-3): 163-165.
- Lauren MH, Hanna SV, Qian S, Frederik FS, Björn S, et al. (2019) Increased glymphatic influx is correlated with high EEG delta power and low heart rate in mice under anesthesia. Sci Adv 5(2): eaav5447.
- Jeffrey JI, Minghuan Wang, Yonghong Liao, Benjamin A Plogg, Weiguo Peng, et al. (2012) A paravascular pathway facilitates CSF flow through the brain parenchyma and the clearance of interstitial solutes, including amyloid b. Sci Transl Med 4(147): 147ra111.
- Benveniste H, Liu X, Koundal S, Sanggaard S, Lee H, et al. (2019) The glymphatic system and waste clearance with brain aging: a review. Gerontology 65(2): 106-119.
- Bjorness TE, Kelly CL, Gao T, Poffenberger V, Greene RW (2009) Control and function of the homeostatic sleep response by adenosine A1 receptors. J Neurosci 29(5): 1267-1276.
- Duffy JF, Zitting KM, Chinoy ED (2015) Aging and circadian rhythms. Sleep medicine clinics 10(4): 423-434.
- Bliwise DL, Ansari FP, Straight LB, Parker KP (2005) Age changes in timing and 24-hour distribution of self-reported sleep. The American journal of geriatric psychiatry 13(12): 1077-1082.
- Homolak J, Mudrovcic M, Vukic B, Toljan K (2018) Circadian rhythm and Alzheimer’s disease. Medical Sciences 6(3): 52.
- Francis PT (2005) The interplay of neurotransmitters in Alzheimer’s disease. CNS Spectr 10(S18): 6-9.
- Lanari A, Amenta F, Silvestrelli G, Tomassoni D, Parnetti L (2006) Neurotransmitter deficits in behavioural and psychological symptoms of Alzheimer’s disease. Mech Ageing Dev 127(2): 158-165.
- H Ferreira-Vieira T, M Guimaraes I, R Silva F, M Ribeiro F (2016) Alzheimer’s disease: targeting the cholinergic system. Current neuropharmacology 14(1): 101-115.
- Yannick Vermeiren, Jana Janssens, Tony Aerts, Jean-Jacques Martin, Anne Sieben, et al. (2016) Brain serotonergic and noradrenergic deficiencies in behavioral variant frontotemporal dementia compared to early-onset Alzheimer’s disease. J Alzheimers Dis 53(3): 1079-1096.
- Mochizuki T, Crocker A, McCormack S, Yanagisawa M, Sakurai T, et al. (2004) Behavioral state instability in orexin knock-out mice. J Neurosci 24(28): 6291-6300.
- Chunmei Wang, Qinqin Wang, Bingyuan Ji, Yanyou Pan, Chao Xu, et al. (2018) The orexin/receptor system: molecular mechanism and therapeutic potential for neurological diseases. Front Mol Neurosci 11: 220.
- Rolf Fronczek, Sarita van Geest, Marijke Frölich, Sebastiaan Overeem, Freek W C Roelandse, et al. (2012) Hypocretin (orexin) loss in Alzheimer’s disease. Neurobiol Aging 33(8): 1642–1650.
- Frank M Schmidt, Juergen Kratzsch, Hermann-Josef Gertz, Mandy Tittmann, Ina Jahn, et al. (2013) Cerebrospinal fluid melanin-concentrating hormone (MCH) and hypocretin-1 (HCRT1, orexin-A) in Alzheimer’s disease. PLoS ONE 8(5): e63136.
- Michelangelo Maestri, Luca Carnicelli, Gloria Tognoni, Elisa Di Coscio, Filippo Sean Giorgi, et al. (2015) non-rapid eye movement sleep instability in mild cognitive impairment: a pilot study. Sleep Med 16(9): 1139-1145.
- Peter-Derex L, Yammine P, Bastuji H, Croisile B (2015) Sleep and Alzheimer’s disease. Sleep Med Rev 19: 29-38.
- Akiko Satoh, Cynthia S Brace, Gal Ben-Josef, Tim West, David F Wozniak, et al. (2010) SIRT1 promotes the central adaptive response to diet restriction through activation of the dorsomedial and lateral nuclei of the hypothalamus. J Neurosci 30(30): 10220-10232.
- Gais S, Born J (2004) Declarative memory consolidation: mechanisms acting during human sleep. Learning & Memory 11(6): 679-685.
- Gareri P, Castagna A, Cotroneo AM, Putignano S, De Sarro G, et al. (2015) The role of citicoline in cognitive impairment: pharmacological characteristics, possible advantages, and doubts for an old drug with new perspectives. Clin Interv Aging 10: 1421-1429.
- Olivia Hurtado, Macarena HJ, Juan G Zarruk, María I Cuartero, Iván Ballesteros, et al. (2013) Citicoline (CDPcholine) increases S irtuin1 expression concomitant to neuroprotection in experimental stroke. J Neurochem 126(6): 819-826.
- Li DJ, Huang F, Ni M, Fu H, Zhang LS, et al. (2016) a7 Nicotinic Acetylcholine Receptor Relieves Angiotensin II-Induced Senescence in Vascular Smooth Muscle Cells by Raising Nicotinamide Adenine Dinucleotide-Dependent SIRT1 Activity. Arterioscler Thromb Vasc Biol 36(8): 1566-1576.
- Rodrigo Medeiros, Nicholas A Castello, David Cheng, Masashi Kitazawa, David Baglietto-Vargas, et al. (2014) a7 Nicotinic receptor agonist enhances cognition in aged 3xTg-AD mice with robust plaques and tangles. The American journal of pathology 184(2): 520-529.
- Steriade MM, McCarley RW (2013) Brainstem control of wakefulness and sleep. Springer Science & Business. Media.
- Ludwig Trillo, Devsmita Das, Wayne Hsieh, Brian Medina, Sarah Moghadam, et al. (2013) Ascending monoaminergic systems alterations in Alzheimer’s disease. Translating basic science into clinical care. Neurosci Biobehav Rev 37(8): 1363-1379.
- Sergiy Libert, Kelli Pointer, Eric L Bell, Abhirup Das, Dena E Cohen, et al. (2011) SIRT1 activates MAO-A in the brain to mediate anxiety and exploratory drive. Cell 147(7): 1459-1472.
- Chaudhuri AD, Yelamanchili SV, Fox HS (2013) MicroRNA-142 reduces monoamine oxidase A expression and activity in neuronal cells by downregulating SIRT1. PLoS ONE 8(11): e79579.
- Burdakov D, Karnani MM, Gonzalez A (2013) Lateral hypothalamus as a sensor-regulator in respiratory and metabolic control. Physiol Behav 121: 117-124.
- Willie JT, Sinton CM, Maratos-Flier E, Yanagisawa M (2008) Abnormal response of melanin-concentrating hormone deficient mice to fasting: hyperactivity and rapid eye movement sleep suppression. Neuroscience 156(4): 819-829.
- Shimada M, Tritos NA, Lowell BB, Flier JS, Maratos-Flier E (1998) Mice lacking melanin-concentrating hormone are hypophagic and lean. Nature 396(6712): 670-674.
- Adamantidis A, de Lecea L (2009) A role for Melanin-Concentrating Hormone in learning and memory. Peptides 30(11): 2066-2070.
- Omar Al-Massadi, Mar Quiñones, Jerome Clasadonte, René Hernandez-Bautista, Amparo Romero-Picó, et al. (2019) MCH Regulates SIRT1/FoxO1 and Reduces POMC Neuronal Activity to Induce Hyperphagia, Adiposity, and Glucose Intolerance. Diabetes 68(12): 2210-2222.
- Nitsan Goldstein, Brian J Levine, Kelsey A Loy, William L Duke, Olivia S Meyerson, et al. (2018) Hypothalamic neurons that regulate feeding can influence sleep/wake states based on homeostatic need. Current Biology 28(23): 3736-3747. e3733.
- Porkka-Heiskanen T, Strecker RE, Thakkar M, Bjørkum AA, Greene RW, et al. (1997) Adenosine: a mediator of the sleep-inducing effects of prolonged wakefulness. Science 276(5316): 1265-1268.
- Porkka-Heiskanen T, Kalinchuk A, Alanko L, Urrila A, Stenberg D (2003) Adenosine, energy metabolism, and sleep. The Scientific World Journal 3: 790-798.
- Benington JH, Kodali SK, Heller HC (1995) Stimulation of A1 adenosine receptors mimics the electroencephalographic effects of sleep deprivation. Brain Res 692(1-2): 79-85.
- Zhi-Li Huang, Wei-Min Qu, Naomi Eguchi, Jiang-Fan Chen, Michael A Schwarzschild, et al. (2005) Adenosine A 2A, but not A 1, receptors mediate the arousal effect of caffeine. Nat Neurosci 8(7): 858-859.
- Muheim C, Brown SA (2013) Adenosine and other purinergic products in circadian timing. In: Adenosine Springer: 213-232.
- Martins I (2017) Sirtuin 1 and adenosine in brain disorder therapy. J Clin Epigenet 3(1).
- Vossel KA, Tartaglia MC, Nygaard HB, Zeman AZ, Miller BL (2017) Epileptic activity in Alzheimer’s disease: causes and clinical relevance. The Lancet Neurology 16(4): 311-322.
- Rebecca L Williams-Karnesky, Ursula S Sandau, Theresa A Lusardi, Nikki K Lytle, Joseph M Farrell, et al. (2013) Epigenetic changes induced by adenosine augmentation therapy prevent epileptogenesis. J Clin Investig 123(8): 3552-3563.
- Lucrezia Cellai, Kevin Carvalho, Emilie Faivre, Aude Deleau, Didier Vieau, et al. (2018) The Adenosinergic Signaling: A Complex but Promising Therapeutic Target for Alzheimer’s Disease. Front Neurosci 12: 520.
- Zisapel N (2007) Sleep and sleep disturbances: biological basis and clinical implications. Cell Mol Life Sci 64(10): 1174-1186.
- Zisapel N (2018) New perspectives on the role of melatonin in human sleep, circadian rhythms and their regulation. Br J Pharmacol 175(16): 3190-3199.
- Tresguerres JA, Kireev R, Tresguerres AF, Borras C, Vara E, et al. (2008) Molecular mechanisms involved in the hormonal prevention of aging in the rat. The Journal of steroid biochemistry and molecular biology 108(3-5): 318-326.
- Zhou JN, Liu RY, Kamphorst W, Hofman MA, Swaab DF (2003) Early neuropathological Alzheimer’s changes in aged individuals are accompanied by decreased cerebrospinal fluid melatonin levels. J Pineal Res 35(2): 125-130.
- Ying-Hui Wu, Matthijs GP Feenstra, Jiang-Ning Zhou, Rong-Yu Liu, Javier Sastre Toranõ, et al. (2003) Molecular changes underlying reduced pineal melatonin levels in Alzheimer disease: alterations in preclinical and clinical stages. The Journal of clinical endocrinology & metabolism 88(12): 5898-5906.
- Sharon L Naismith, Ian B Hickie, Zoe Terpening, Shanthakumar M Rajaratnam, John R Hodges, et al. (2014) Circadian misalignment and sleep disruption in mild cognitive impairment. J Alzheimers Dis 38(4): 857-866.
- Wu YH, Fischer DF, Swaab DF (2007) A promoter polymorphism in the monoamine oxidase A gene is associated with the pineal MAOA activity in Alzheimer’s disease patients. Brain Res 1167: 13-19.
- Tajes M, Gutierrez-Cuesta J, Ortuno-Sahagun D, Camins A, Pallas M (2009) Anti-aging properties of melatonin in an in vitro murine senescence model: involvement of the sirtuin 1 pathway. J Pineal Res 47(3): 228-237.
- Jiraporn Panmanee, Chutikorn Nopparat, Napapit Chavanich, Mayuri Shukla, Sujira Mukda, et al. (2015) Melatonin regulates the transcription of betaAPP-cleaving secretases mediated through melatonin receptors in human neuroblastoma SH-SY5Y cells. J Pineal Res 59(3): 308-320.
- Mukda S, Panmanee J, Boontem P, Govitrapong P (2016) Melatonin administration reverses the alteration of amyloid precursor protein-cleaving secretases expression in aged mouse hippocampus. Neurosci Lett 621: 39-46.
- Chang HM, Wu UI, Lan CT (2009) Melatonin preserves longevity protein (sirtuin 1) expression in the hippocampus of total sleep-deprived rats. J Pineal Res 47(3): 211-220.
- Tresguerres JA, Cuesta S, Kireev RA, Garcia C, Acuña-Castroviejo D, et al. (2013) Beneficial effect of melatonin treatment on age-related insulin resistance and on the development of type 2 diabetes. Hormone molecular biology and clinical investigation 16(2): 47-54.
- Cuesta S, Kireev R, García C, Rancan L, Vara E, et al. (2013) Melatonin can improve insulin resistance and aging-induced pancreas alterations in senescence-accelerated prone male mice (SAMP8). Age 35(3): 659-671.
- Kireev RA, Vara E, Viña J, Tresguerres JA (2014) Melatonin and oestrogen treatments were able to improve neuroinflammation and apoptotic processes in dentate gyrus of old ovariectomized female rats. Age 36(5): 9707.
- Berson DM (2003) Strange vision: ganglion cells as circadian photoreceptors. Trends Neurosci 26(6): 314-320.
- Turner PL, Mainster MA (2008) Circadian photoreception: ageing and the eye’s important role in systemic health. Br J Ophthalmol 92(11): 1439-1444.
- Turner PL, Van Someren EJ, Mainster MA (2010) The role of environmental light in sleep and health: effects of ocular aging and cataract surgery. Sleep Med Rev 14(4): 269-280.
- Vandewalle G, Maquet P, Dijk DJ (2009) Light as a modulator of cognitive brain function. Trends in cognitive sciences 13(10): 429-438.
- Sletten TL, Revell VL, Middleton B, Lederle KA, Skene DJ (2009) Agerelated changes in acute and phase-advancing responses to monochromatic light. J Biol Rhythms 24(1): 73-84.
- Revell VL, Debra J (2010) Impact of age on human non-visual responses to light. Sleep and biological rhythms 8(2): 84-94.
- Carol Yim-Lui Cheung, Yi Ting Ong, M Kamran Ikram, Shin Yeu Ong, Xiang Li, et al. (2014) Microvascular network alterations in the retina of patients with Alzheimer’s disease. Alzheimer’s & Dementia 10(2): 135-142.
- Nazanin Mirzaei, Haoshen Shi, Mia Oviatt, Jonah Doustar, Altan Rentsendorj, et al. (2020) Alzheimer’s Retinopathy: Seeing Disease in the Eyes. Front Neurosci 14: 921.
- Carolina Jaliffa, Ilhame Ameqrane, Anouk Dansault, Julia Leemput, Véronique Vieira, et al. (2009) Sirt1 involvement in rd10 mouse retinal degeneration. Invest Ophthalmol Vis Sci 50(8): 3562-3572.
- Shawn C Maloney, Emilia Antecka, Alexandre N Odashiro, Bruno F Fernandes, Madeline Doyle, et al. (2012) Expression of SIRT1 and DBC1 in developing and adult retinas. Stem cells international: 908183.
- Hwei-Ling Cheng, Raul Mostoslavsky, Shinichi Saito, John P Manis, Yansong Gu, et al. (2003) Developmental defects and p53 hyperacetylation in Sir2 homolog (SIRT1)-deficient mice. Proceedings of the National Academy of Sciences 100(19): 10794-10799.
- Chen D, Pacal M, Wenzel P, Knoepfler PS, Leone G, et al. (2009) Division and apoptosis of E2f-deficient retinal progenitors. Nature 462(7275): 925-929.
- Yi J, Luo J (2010) SIRT1 and p53, effect on cancer, senescence and beyond. Biochimica et Biophysica Acta (BBA)-Proteins and Proteomics 1804(8): 1684-1689.
- Hui Zhang, Seong-Hoon Park, Brooke G Pantazides, Oleksandra Karpiuk, Matthew D Warren, et al. (2013) SIRT2 directs the replication stress response through CDK9 deacetylation. Proc Natl Acad Sci 110(33): 13546-13551.
- Norimitsu Ban, Yoko Ozawa, Takaaki Inaba, Seiji Miyake, Mitsuhiro Watanabe, et al. (2013) Light–dark condition regulates sirtuin mRNA levels in the retina. Exp Gerontol 48(11): 1212-1217.
- Anthony E Civitarese, Stacy Carling, Leonie K Heilbronn, Mathew H Hulver, Barbara Ukropcova, et al. (2007) Calorie restriction increases muscle mitochondrial biogenesis in healthy humans. PLoS Med 4(3): e76.
- Heilbronn LK, Civitarese AE, Bogacka I, Smith SR, Hulver M, et al. (2005) Glucose tolerance and skeletal muscle gene expression in response to alternate day fasting. Obes Res 13(3): 574-581.
- Zhihui Yin, Demin Gao, Ke Du, Chen Han, Yuhan Liu, et al. (2022) Rhein Ameliorates Cognitive Impairment in an APP/PS1 Transgenic Mouse Model of Alzheimer’s Disease by Relieving Oxidative Stress through Activating the SIRT1/PGC1alpha Pathway. Oxid Med Cell Longev: 2524832.
- Arunsundar M, Shanmugarajan TS, Ravichandran V (2015) 3,4dihydroxyphenylethanol attenuates spatio-cognitive deficits in an Alzheimer’s disease mouse model: modulation of the molecular signals in neuronal survival-apoptotic programs. Neurotox Res 27(2): 143-155.
- R Scott Turner, Ronald G Thomas, Suzanne Craft, Christopher H van Dyck, Jacobo Mintzer, et al. (2015) A randomized, double-blind, placebo-controlled trial of resveratrol for Alzheimer disease. Neurology 85(16): 1383-1391.
- Lee J, Torosyan N, Silverman DH (2017) Examining the impact of grape consumption on brain metabolism and cognitive function in patients with mild decline in cognition: A double-blinded placebo-controlled pilot study. Exp Gerontol 87(Pt A): 121-128.
- Charbel Moussa, Michaeline Hebron, Xu Huang, Jaeil Ahn, Robert A Rissman, et al. (2017) Resveratrol regulates neuroinflammation and induces adaptive immunity in Alzheimer’s disease. J Neuroinflammation 14(1): 1.
- Abozaid OAR, Sallam MW, El-Sonbaty S, Aziza S, Emad B, et al. (2022) Resveratrol-Selenium Nanoparticles Alleviate Neuroinflammation and Neurotoxicity in a Rat Model of Alzheimer’s Disease by Regulating Sirt1/miRNA-134/GSK3beta Expression. Biol Trace Elem Res 200(12): 5104-5114.
- Younes S (2024) The efficacy of a 24-hour preoperative pause for SGLT2inhibitors in type II diabetes patients undergoing bariatric surgery to mitigate euglycemic diabetic ketoacidosis, Diabetes Epidemiology and Management 14: 100201.
- Younes S (2024) The role of nutrition on the treatment of Covid 19. Human Nutrition & Metabolism 36: 200255.
- Younes S (2024) The role of micronutrients on the treatment of diabetes. Human Nutrition & Metabolism 35: 200238.
- Younes S (2023) The impact of micronutrients on the sense of taste. Human Nutrition & Metabolism 35: 200231.
- Younes Sand Shbani A (2024) The influence of diabetes on micro albunimuria. Int J Endocrinol Diabetes 7(1): 166.
- Younes S (2024) The impact of b-cell Replication on Diabetes Therapy. Int J Endocrinol Diabetes 7(1): 167.
- Samer Y (2024) Gender Difference in Nutritional Knowledge, Dietary Pattern and Nutritional Status of Undergraduates in Tartous University, Syria. Nutri Food Sci Int J 13(2): 555857.
- Samer Younes (2024) The Implications of Pyroptosis in Conditions Affecting the Genitourinary Tract. Annals of Urology & Nephrology 4(3).
- Younes S (2024) A Comprehensive Examination of the Nutritional Sufficiency of Vegan Recipes Widely Available in the Market. J. Nutrition and Food Processing 7: 5.
-
Samer Younes*. The Influence of Sleep on Brain Health. Glob J Aging Geriatr Res. 3(1): 2024. GJAGR.MS.ID.000555.
-
Slow Wave Sleep, Alzheimer's Disease (AD), Brain Health, Sleep Regulation, Sirtuins, NREM Sleep, Retinoic Acid, Amyloid Precursor Protein, Sleep Disorders
-
This work is licensed under a Creative Commons Attribution-NonCommercial 4.0 International License.