Review Article
Method: Efficient Ocular Drug Delivery Using Fluorinated Nanoparticle Laden Films
Sina Dehestani*
Department of Pharmaceutical Sciences, University of Pittsburgh, Pittsburgh, 15261, USA
Sina Dehestani, Department of Pharmaceutical Sciences, University of Pittsburgh, Pittsburgh, 15261, USA
Received Date:February 02, 2024; Published Date:February 09, 2024
Introduction
Glaucoma is a chronic disease that affected approximately 61.0 million people worldwide in 2010 [1]. The number is predicted to rise approximately to 76.0 million by 2020 and 112.0 million by 2040 [2]. Glaucoma is the most common cause of vision loss worldwide and is the second most common ocular disease after cataracts [3]. This disease is usually diagnosed in the advanced disease stages when vision is seriously impaired. However, conventional formulations such as solutions and ointments are not beneficial due to poor ocular bioavailability (<5%), despite being patient compliant and non-invasive [4]. The poor retention time of the drugs requires patients to instill eye drops frequently, increasing the possibility of low adherence to the treatment. There are several barriers in the ocular delivery system that prevent therapeutic agents from achieving the desired concentration at the target sites for a prolonged duration. Reflex blinking (5-7 blinks/min), tear turnover (0.5-2.2 μL/min), protein binding, and nasolacrimal duct elimination cause poor retention time and removes approximately 90% of the dose administered via topical instillation [5]. The muco–aqueous barrier on the tear film protects the anterior surface of the eye from particles entering [6]. The epithelial and endothelial membranes are hydrophobic, while the stroma is hydrophilic and forms up to 90% of the corneal thickness. This distinctive hydrophobic/hydrophilic/ hydrophobic anatomy prevents small molecules from passing through the cornea [5]. Tight junctions expressed in the epithelial and endothelial layers prevent particles from passing across the cornea via the paracellular pathways. Also, multiple layers of the corneal epithelium (5-7 layers) make it difficult for drugs to cross the anterior segment of the cornea via the transcellular pathway [7]. The presence of various metabolic enzymes in the ocular tis sues including peptidases, carbonic anhydrases, N-dealkylating enzyme, and esterase poses another challenge for drug delivery [8]. The short retention time of the drugs in posterior ocular tissues reduces the therapeutic efficacy [9]. Various peptides, proteins, and glycoproteins present in the tear film could non-specifically bind to nanocarriers or drugs [10]. Vitreous humor contains dense collagen and glycosaminoglycans which can interfere with large molecules and prevent the diffusion of nanocarriers [11].
Cell penetrating peptides (CPPs) have been widely studied for ocular drug delivery. Their potential to deliver therapeutic agents across the posterior tissues has been demonstrated in several studies. Liu et al. used penetratin as a penetration enhancer for ocular drug delivery via eye drop instillation [12]. Within 10 min of administration, penetratin-functionalized carrier was able to enter both anterior and posterior parts of the eye. It was distributed widely in all layers of retina-choroid and up taken by the retinal pigment epithelium (RPE). Accumulation peaked at 30 min and was detectable for more than 6 h after instillation, showing extended retention in these tissues. Li et al. used TAT combined arginine-glycine-aspartic (RGD) as a novel penetration enhancer for ocular drug delivery by eye drop [13]. 14-fold higher concentrations of TAT-RGD carriers were detected by ELISA in the retina at 1 h compared to the control groups. Accumulation peaked at around 60 min and their half-lives average was 9.64 ± 1.31 h. de Cogan et al. studied the delivery of anti-VEGF drugs using poly-arginine based CPP: (5-carboxyfluorescein- RRRRRR-COOH) for ocular penetration via eye drop application [14]. Drug accumulation in the retina peaked at 40 min after administration containing 0.2% of the applied dose, followed by elimination in 24 h. Despite CPP-mediated drug delivery showing great potential to deliver drugs in the posterior eyes, the delivery mechanism is still unknown [15]. More importantly, several issues need to be addressed when using CPP as a penetrating enhancer. The nonspecific binding of negatively charged molecules such as albumin to CPPs could reduce the CPP-membrane interactions, resulting in poor internalization [16]. (2) 99% actively delivered cargoes are entrapped in the endosome which prevents them from penetrating multiple corneal epithelium layer [17]. CPP-functionalized nanocarriers are usually cytotoxic because they puncture through membranes that disrupt membrane structure [18]. (4) The negatively charged proteoglycans in the vitreous humor and sclera could interact with positively charged CPPs, resulting in poor permeability [11].
Recently, fluoroamphiphiles were reported to have promising features, efficient drug delivery, high mucopenetration, and imaging feasibility. Fluorocarbons could improve the affinity of polymers to cell membranes and facilitate endocytosis [19]. In addition, the fluorous molecules are lipophobic and hydrophobic simultaneously and this property makes them bioinert to avoid nonspecific binding by endogenous molecules and the protection of loaded drugs [20]. Finally, amphiphiles with fluoroalkyls could reduce cytotoxicity compared with conventional micelles [20]. A study published on Nature Communications demonstrated that the use of fluoroamphiphiles for cytosolic protein delivery showed advantages over other existing methods [21]. Li et al. reported that fluorocarbon-modified carriers could achieve more than 90% of gene transfection for a CRISPR-Cas-9 system and an approximately 100% cellular uptake rate [22]. Beside their improved drug delivery efficiency, fluoroamphiphiles exhibited strong mucopenetration ability. For example, Ge et al. found that fluorination of the polypeptides can drastically enhance the mucus permeation ability by 240 folds, suggesting fluoroamphiphile could be a powerful candidate for mucopenetrating applications [23]. Finally, fluoroamphiphiles could be used as a contrast agent for magnetic resonance imaging (MRI). The [19] F nucleus has a 100% natural abundance and negligible endogenous fluorine offers [19] F MRI with high contrast-to-noise ratio and specificity [24]. Both perfluoropolyether (PFPE, a perfluorinated polyethylene glycol) and perfluoro‐15‐crown‐5‐ether (PFCE) are used for [19] F MRI because of high sensitivity and unified major fluorine peaks [25,26]. A comprehensive study [27] revealed that the bioaccumulation of seven or fewer fluorinated carbons is several orders of magnitudes lower than “legacy” compounds classified as bioaccumulative. Moreover, the presence of ether and methyl groups in the fluorocarbons endows them with biodegradation properties. Taken together, our solution is to engineer a fluorinated nanoparticle based on PFPE for drug delivery because of its high sensitivity in [19] F MRI and biodegradable properties.
In this study, we developed a fluorinated nanoparticle laden film to deliver first-line drug, travoprost, into ocular tissues to treat glaucoma (Figure 1). Our film consists of a combination of clinically used polymers (Eudragit® E PO, FS 30 D, HPMC, and HEC 250 L) and mucoadhesive PDA. The adhesion mechanism of this film relies under both physical association and covalent bonding between PDA and mucus. Micelles composed of CPP-modified¬ polyethylene glycol-b-polyperfluoroether (CPP-PEG- PFPE) and (pentafluoroethyl) polyethylene glycol-b-polyperfluoroether (C2F5-PEG-PFPE) are incorporated into the film (Figure 2). Travoprost is loaded in the micelles via a solvent evaporation method. We hypothesize that pentafluoroethyl groups could not incorporate into the PFPE core because fluorophilic effect of two fluorocarbons is too weak to self-assembly [28] and the steric effect of PEG prevents pentafluoroethyl groups from interacting with the core while it still could repel positively charged CPPs on the surface. Here, RGD improves micelle-membrane interactions. Fluorocarbon structure endows nanocarrier with enhanced membrane permeability. Surface-modified fluorocarbon prevents adsorption of mucin glycoproteins and improves mucopenetration ability. This nanoparticle could tackle several issues in CPP-mediated drug delivery: (1) Surface-modified fluorocarbon could minimize the non-specific binding of negatively charged molecules such as albumin to CPPs, maintaining delivery efficiency of CPPs. Also, it can increase the local concentration of peptides that can interact with the membrane via its bioinert property and strong hydrophobicity, causing improved penetration capacity (Figure 3). As shown in (Figure 4), the cluster of fluorocarbons could be observed by TEM because of the high electron density of fluorine [29]. (2) By utilizing the unique hydrophobic and lipophobic behaviors, fluorocarbon could help the drug carrier traverse the lipid bilayers of cells including the endosome/lysosome membrane, facilitating endosomal escape. Also, due to high stability and chemical inertness, fluorinated nanoparticles could protect entrapped drugs from enzymatic degradation. (3) Fluorinated particles have stable structure and special hydro/lipophobic property that minimize the interactions between particles and the membrane, leading to reduced cell-membrane disruption and efficient membrane penetration [30]. (4) Fluorocarbon could effectively prevent CPP-functionalized particles from adhering to the proteoglycans. The combination of mucopenetrating fluorinated nanoparticles and mucoadhesive films has great promise to address most of the issues in ocular drug delivery. (1) The mucoadhesive film adhering to the mucin in the tear film protects nanoparticles from being washed by tear and prolongs the retention time of drug carriers. Also, it can enhance the contact between nanocarriers and mucins. (2) The highly mucopenetrating fluorinated nanoparticles could cross mucin and reach the layer. (3) The soluble fluorinated nanoparticles can pass through the hydrophobic membranes effectively via the strong concentrated CPP-membrane interactions and bioinert fluorocarbon. Moreover, the ability of fluorinated particles to penetrate the membrane could overcome the hurdles of crossing multiple epithelial layers. Thus, our nanocarrier could freely cross both hydrophobic and hydrophilic barriers. (4) The inertness of fluorocarbon could avoid non-specific binding of various biomolecules, prevent interactions with glycosaminoglycans in the vitreous humor, and protect loaded drugs from being degraded by enzymes. (5) The strong fluorophilic effects formed in PFPE cluster offer our nanoparticle high stability that resists extensive dilution in physiological environments. Because of the presence of perfluorocarbon, travoprost can bind to fluorocarbon and prevent burst release (Figure 5). This could address the issue of short retention time of drugs in ocular tissues and achieve a desired pharmacokinetic pro file. Apart from drug delivery, this fluorinated nanoparticle could be utilized as a contrast agent for [19] F MRI. Due to the difference in mobility of [19] F in travoprost and PFPE, it is possible to distinguish the signals from the nanocarrier and the drug. Thus, it will be unprecedented to real-time monitor the in vivo distribution of the drug and its carrier simultaneously and decode the mystery of pharmacokinetic properties of nanoparticles in ocular tissues in a direct manner.
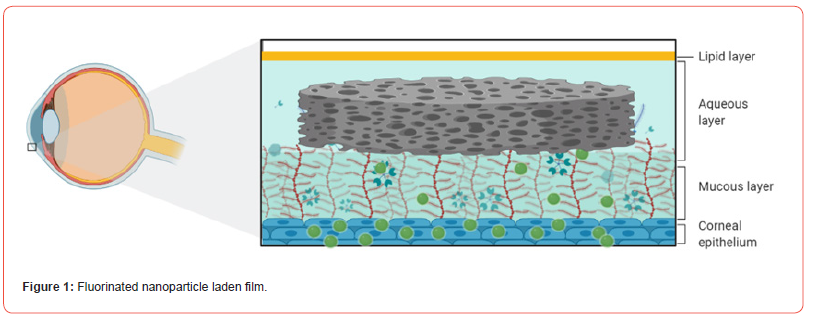
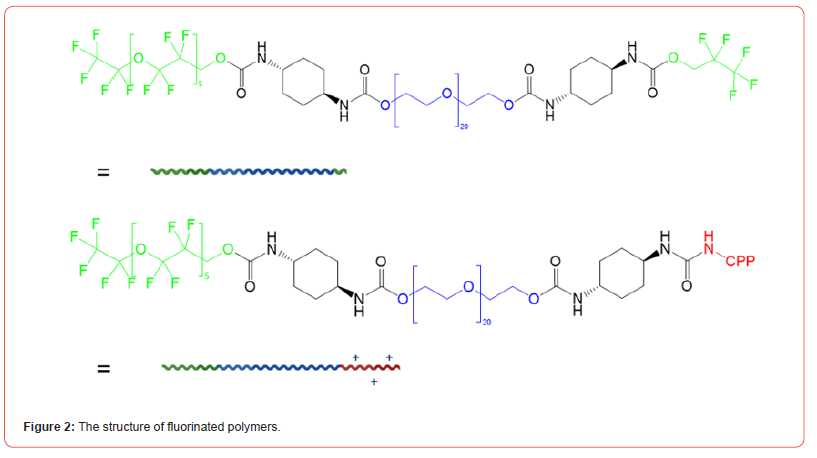
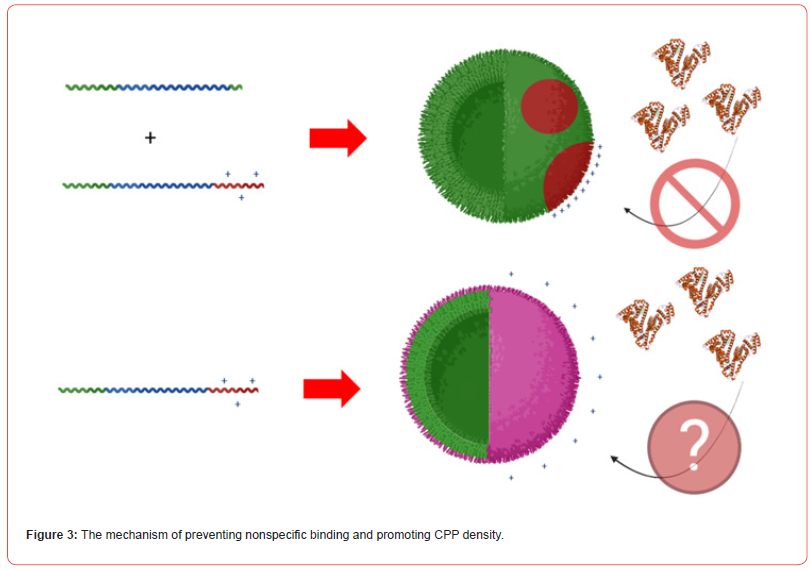
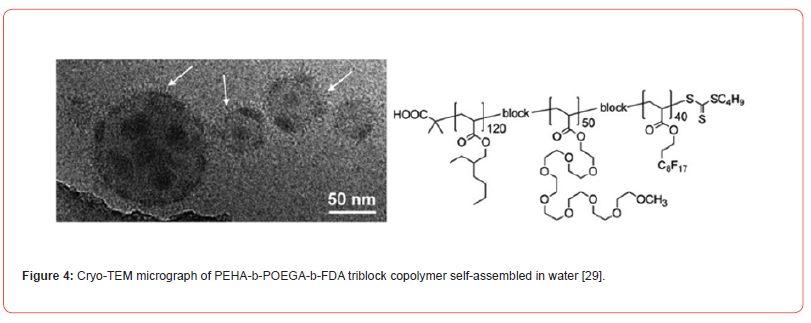
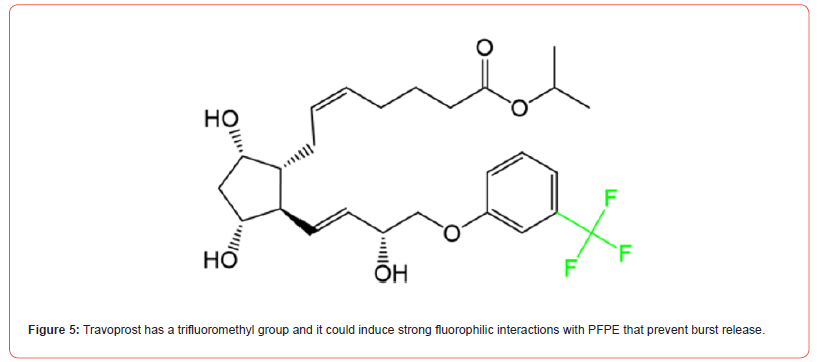
Methods and materials
Synthesis of C2F5-PEG-PFPE
Solvent evaporation method is used to synthesize C2F5-PEGPFPE and CPP-PEG- PFPE 28. Trans-1,4-diisocyanatocyclohexane (0.1 mol) was added into a 200 mL flask equipped with a thermometer and a mechanical stirrer. Then, pentafluoroethanol (0.1 mol) was added drop-wisely into the flask. After the addition of 0.2 g bismuth neodecanoate (AC-83) as a catalyst, the temperature was maintained at 80 °C for 8 h. PEG (0.05 mol) was added and allowed to react for 10 h to yield C2F5-PEG conjunction. Finally, the product was purified by dialysis for 48 h. The same method was used to conjugate C2F5-PEG with PFPE (Scheme 1).
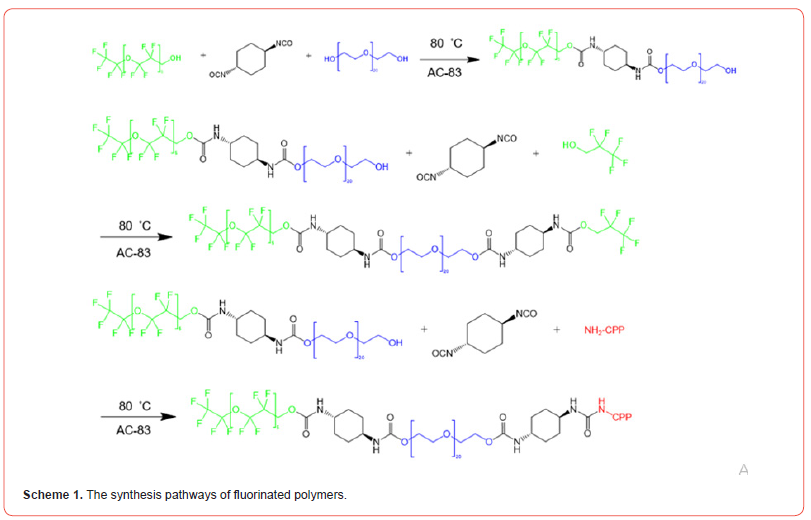
Synthesis of CPP-PEG- PFPE
trans-1,4-diisocyanatocyclohexane (0.05 mol) was added into a 200 mL flask equipped with a thermometer and a mechanical stirrer. Then, CPP (0.05 mol) was added drop-wisely into the flask. After addition of 0.2 g bismuth neodecanoate (AC-83) as a catalyst, the temperature was maintained at 80 °C for 8 h. PEG (0.025 mol) was added and allowed to react for another 10 h to yield CPPPEG conjunction. Finally, the product was purified by dialysis for 48 h. The same method was used to conjugate CPP-PEG with PFPE (Scheme 1).
Preparation of travoprost-loaded nanomicelles
The solvent evaporation method was used to prepare the travoprost- loaded nanomicelles (TLNMs). Next, 100 mg of travoprost and 100 mg of a fluorinated polymeric surfactant (95mg of C2F5- PEG-PFPE and 5mg of CPP-PEG- PFPE, this ratio can be adjusted) were dissolved in 10 mL of tetrahydrofuran (THF), and the conjugate was mixed using magnetic stirrers for 20 min at 300 rpm (the amount of the drug and fluorinated polymeric surfactant can be adjusted to find the maximum loading capacity. After that, the THF was evaporated using a rotary evaporation machine at room temperature with the help of a water pump to obtain an extremely thin and dry layer. Then, the TLNM was received by adding 100 mL of water to the thin dry layer while stirring for over 30 min. The free travoprost was removed by centrifuging the nanomicelles obtained above 8000 rpm for 5 min. The sediment was weighed to calculate the improved solubility and entrapment efficiency (EE%).
Acknowledgement
None.
Conflict of Interest
No Conflict of Interest.
References
- Quigley HA, Broman AT (2006) The number of people with glaucoma worldwide in 2010 and 2020. British journal of ophthalmology 90(3): 262-267.
- Tham Y-C, Li X, Wong TY, Quigley HA, Aung T, et al. (2014) Global prevalence of glaucoma and projections of glaucoma burden through 2040: a systematic review and meta-analysis. Ophthalmology 121(11): 2081-2090.
- Kingman S (2004) Glaucoma is the second leading cause of blindness globally. Bulletin of the World Health Organization 82(11): 887-888.
- Andrés-Guerrero V, Bravo-Osuna I, Pastoriza P, Molina-Martinez IT, Herrero-Vanrell R, et al. (2017) Novel technologies for the delivery of ocular therapeutics in glaucoma. Journal of Drug Delivery Science and Technology 42: 181-192.
- Bachu RD, Chowdhury P, Al-Saedi ZH, Karla PK, Boddu SH, et al. (2018) Ocular drug delivery barriers—role of nanocarriers in the treatment of anterior segment ocular diseases. Pharmaceutics 10(1): 28.
- Diebold Y, Calonge M (2010) Applications of nanoparticles in ophthalmology. Progress in retinal and eye research 29(6): 596-609.
- Vyas S, Kakade P, Chogale M, Disouza J, Patravale V, et al. (2015) Multidimensional ophthalmic nanosystems for molecular detection and therapy of eye disorders. Current pharmaceutical design 21(22): 3223-3238.
- Cholkar K, Patel SP, Vadlapudi AD, Mitra AK (2013) Novel strategies for anterior segment ocular drug delivery. Journal of ocular pharmacology and therapeutics 29(2): 106-123.
- Cheruvu NP, Kompella UB (2006) Bovine and porcine transscleral solute transport: influence of lipophilicity and the Choroid–Bruch’s layer. Investigative ophthalmology & visual science 47(10): 4513-4522.
- Green-Church KB, Nichols KK, Kleinholz NM, Zhang L, Nichols JJ, et al. (2008) Investigation of the human tear film proteome using multiple proteomic approaches. Molecular vision 14: 456-470.
- Mains J, Wilson CG (2013) Vitreous humor as a barrier to nanoparticle distribution. Journal of ocular pharmacology and therapeutics 29(2): 143-150.
- Liu C, Tai L, Zhang W, Wei G, Pan W, et al. (2014) Penetratin, a potentially powerful absorption enhancer for noninvasive intraocular drug delivery. Molecular pharmaceutics 11(4): 1218-1227.
- Yan L, Lian L, Zhiwei L, Juzheng S, Xinke Z, et al. (2016) Tat PTD-Endostatin-RGD: A novel protein with anti-angiogenesis effect in retina via eye drops. Biochimica et Biophysica Acta (BBA)-General Subjects 1860(10): 2137-2147.
- Felicity DC, Lisa JH, Aisling L, Peter JMW, Judith L, et al. (2017) Topical delivery of anti-VEGF drugs to the ocular posterior segment using cell-penetrating peptides. Investigative ophthalmology & visual science 58(5): 2578-2590.
- Thareja A, Hughes H, Alvarez-Lorenzo C, Hakkarainen JJ, Ahmed Z, et a. (2021) Penetration Enhancers for Topical Drug Delivery to the Ocular Posterior Segment—A Systematic Review. Pharmaceutics 13(2): 276.
- Kosuge M, Takeuchi T, Nakase I, Jones AT, Futaki S, et al. (2008) Cellular internalization and distribution of arginine-rich peptides as a function of extracellular peptide concentration, serum, and plasma membrane associated proteoglycans. Bioconjugate chemistry 19(3): 656-664.
- Van Den Berg A, Dowdy SF (2011) Protein transduction domain delivery of therapeutic macromolecules. Current opinion in biotechnology 22(6): 888-893.
- Kauffman WB, Fuselier T, He J, Wimley WC (2015) Mechanism matters: a taxonomy of cell penetrating peptides. Trends in biochemical sciences 40(12): 749-764.
- Riess JG (2009) Highly fluorinated amphiphilic molecules and self-assemblies with biomedical potential. Current opinion in colloid & interface science 14(5): 294-304.
- Santaella C, Vierling P, Riess J (1992) New Perfluoroalkylated Phospholipids as Injectable Surfactants: Synthesis, Preliminary Physicochemical, and Biocompatibility Data. ChemInform 20(2-4): 835-837.
- Zhang Z, Shen W, Ling J, Yan Y, Hu J, et al. (2018) The fluorination effect of fluoroamphiphiles in cytosolic protein delivery. Nature communications 9: 1-8.
- Ling L, Linjiang S, Xiaowei L, X Yang, Xia L, et al. (2017) Artificial virus delivers CRISPR-Cas9 system for genome editing of cells in mice. ACS nano 11(1): 95-111.
- Ge C, Yang J, Duan S, Liu Y, Meng F, et al. (2020) Fluorinated α-helical polypeptides synchronize mucus permeation and cell penetration toward highly efficient pulmonary siRNA delivery against acute lung injury. Nano letters 20(3): 1738-1746.
- Ruiz‐Cabello J, Barnett BP, Bottomley PA, Bulte JW (2011) Fluorine (19F) MRS and MRI in biomedicine. NMR in Biomedicine 24(2): 114-129.
- Janjic JM, Srinivas M, Kadayakkara DK, Ahrens ET (2008) Self-delivering nanoemulsions for dual fluorine-19 MRI and fluorescence detection. Journal of the American Chemical Society 130(9): 2832-2841.
- Ahrens ET, Flores R, Xu H, Morel PA (2005) In vivo imaging platform for tracking immunotherapeutic cells. Nature biotechnology 23: 983-987.
- Conder JM, Hoke RA, Wolf Wd, Russell MH, Buck RC, et al. (2008) Are PFCAs bioaccumulative? A critical review and comparison with regulatory criteria and persistent lipophilic compounds. Environmental science & technology 42(4): 995-1003.
- Wei B (2021) Fluorinated Polymeric Surfactant with a Pluronic-like Structure and Its Application as a Drug Carrier. ACS Applied Polymer Materials 3: 4940-4948.
- Berlepsch Hv, Böttcher C, Skrabania K, Laschewsky A (2009) Complex domain architecture of multicompartment micelles from a linear ABC triblock copolymer revealed by cryogenic electron tomography. Chemical communications: 2290-2292.
- Dowdy SF (2017) Overcoming cellular barriers for RNA therapeutics. Nature biotechnology 35(3): 222-229.
-
Sina Dehestani*. Method: Efficient Ocular Drug Delivery Using Fluorinated Nanoparticle Laden Films. Arch Phar & Pharmacol Res. 4(1): 2024. APPR.MS.ID.000578.
-
Drug Delivery, Fluorinated Nanoparticle, Corneal Thickness, Glaucoma, Fluorocarbons, Nanomicelles
-
This work is licensed under a Creative Commons Attribution-NonCommercial 4.0 International License.