Review Article
Climate Variability over Southern Africa and Implications for Water Resources: A Review
Sibongakonke BH Hadebe and Lawrence T Dube*
Discipline of Geography, School of Agricultural, Earth and Environmental Sciences, University of KwaZulu-Natal, Pietermaritzburg 3201, South Africa
Lawrence T Dube, Discipline of Geography, School of Agricultural, Earth and Environmental Sciences, University of KwaZulu-Natal, Pietermaritzburg 3201, South Africa
Received Date: November 23, 2023; Published Date: January 05, 2024
Abstract
The African continent is highly sensitive to climate change and variability due to its low adaptive capacity. Extreme weather events, such as droughts, and floods, have been occurring with a higher degree of frequency over the last two decades in southern Africa. The review synthesizes existing literature on the various aspects of climate variability, including changes in temperature, rainfall patterns, and extreme weather events in the region, with particular emphasis over South Africa. It also explores the effects of variations in climate on water availability and quality. The spatial and temporal aspects of rainfall and temperature are explored. Rainfall variability over southern Africa has been linked to the ENSO, regional circulations, and other synoptic features. The hydrological cycle has been found to intensify in response to temperature increases because of an increase in both rainfall and evaporation. This paper reviews the existing body of knowledge on climate variability and impacts on water resources in South Africa using recent and older literature in order to gain a continuum in the review. Understanding climate change and variability is crucial for effective water resource management. It allows anticipation and preparations for potential water shortages, floods, and other water-related hazards. This information is critical for making informed decisions about water allocation, infrastructure development, and conservation strategies.
Keywords:Climate variability and change; ENSO; drought; floods; temperature; rainfall
Introduction
Africa is a highly vulnerable region to climate change and variability because of its low adaptive capacity [1,2]. Southern Africa is characterized as a water-scarce region and is rated as one of the most prone to climate variability and change in Africa [3]. Climate change and variability have a direct as well as an indirect impact on the water resources, biophysical ecosystems, agriculture, socio-economy and energy generation [4-8]. According to the IPCC [9], the effects of climate variability on water resources is expected to be more extreme than previously predicted. Several studies have noted that there is certainty that any changes in climate will affect the use of water and its availability [6,7,10-12]. The region of southern Africa is highly vulnerable to the impacts of climate variability and change and is projected to experience severe rainfall shortages in the coming decades [13]. However, with the best of our knowledge, there is a shortage of studies assessing the responses of water resources to climate variability in southern Africa [14-16]. The expected changes and variations in climate in Southern Africa include an increase in both maximum and minimum temperatures, drying conditions and the intensity and frequency of extreme weather events [17,18]. Variations in the frequency and intensity of extreme climatic events, as well as changes in weather pattern variability, have profound implications for various environmental sectors such as water, soil, biodiversity, and agriculture [19]. Extreme weather events such as heatwaves, drought, and floods events are likely to become more frequent and intense for the balance of the century [1]. These events are driven by Ocean- Atmospheric interactions such as El Niño-Southern Oscillation (ENSO), which have numerous negative consequences beyond the impacts caused by climate variability and climate changes in mean annual rainfall and temperature [20]. ENSO have been recognized as one of the major causes of rainfall variability in southern Africa, making it a crucial factor of floods and droughts occurrence in this region [19,21-23]. The link between ENSO and rainfall across southern Africa has been demonstrated to be substantial, however a few uncertainties remain [21]. Rainfall variability in southern Africa, is not always attributable to the ENSO effect since the oceanatmosphere interaction is additionally affected by the existence of other weather phenomena [19] such as Southern Annular Mode (SAM) during either El Niño or La Niña phases [24].
Climate Change Over Southern Africa
For the past 65 years, the world has recorded significant changes in climate [25]. Climate change has an effect on different elements of the ecological, environmental, socio-economic and sociopolitical disciplines [26,27]. According to the IPCC (AR4), there has been evidence of increase in climate variability globally, associated with the warming of the earth (Thornton et al. 2014). Such changes in frequency of extreme events and variation in weather patterns are projected to have a significant impact on humans and natural systems. The IPCC’s sixth Assessment Report (AR6) (2021) [9] suggests an increased in frequency of heavy rainfalls over South Africa in the future. The increase in the frequency of extreme events such as heat waves, floods and droughts has been projected for the entire century [28-30]. Such events will have adverse impacts on natural systems, including hydrological resources.
Ibebuchi [31] reported that the 1992/93 drought posed significant impacts in southern Africa, especially in South Africa. Ngoran et al. [32], and Dube and Jury [14], observed that 1992/93 drought resulted in major challenges on water resources, decreasing water quality and quantity The implications of climate change and variability in southern Africa are exacerbated by the fact that the region Africa is largely dependent on agriculture which constitutes an average 35% of the GDP of the Member States and over 70 percent of employment in the region (SADC Multi-country Agricultural Productivity Programme (SADC MAPP) [33]. A 10% decline in rainfall results in a 5% and 0.05% decline in crop yield and GDP, respectively, in South Africa [34]. [9,35,36] IPCC (2021), reported a decrease in agricultural production of 34% in Africa since 1961 as the result of climate change. A study by Dube and Jury [14] reported the impacts of the 1992/93 drought in KwaZulu- Natal (KZN), South Africa, using dam inflows and crop yield. In this study, the 1991/93 dam level inflow of Midmar dam decreased from 108 m3 before the onset of the drought to 106 m3 during 1993. The drought which became a dominant feature of climate variability at the time, lead to the decline in agricultural production in KZN from 3 T.ha-1, the potential yield, to 0.85 T.ha-1. Ndlovu and Demlie [37] observed that the 2014/15 drought in KZN, caused the storage levels of dams to decline to less than 50% of their capacity. For example, the Hluhluwe dam was observed to be at 22% of its normal level because of the water inflow reduction from 280 to 90 m3.h-1 and this caused water usage restrictions to increase from 15% to 50%. Bukhosini and Moyo [38], observed that the 2014 – 16 drought affected small scale farmers in a way that 22% of the farmers suffered livestock deaths and body weight loss for those that survived, and 24% suffered crop failure in Mtubatuba (KZN).
South Africa is also vulnerable to flood events which influence the quality of water resources [39] and bears adverse health impacts through water-borne diseases [39-41]. Ndlovu and Demlie [37] studied the meteorology of extreme wet events of 1984 and 2000 associated with tropical cyclones Demonia and Eline, respectively. The devastation caused by these events is well documented [42- 44]. Other extreme wet events include the 1999/2000 La Niña year and its significant impact on water quality documented in Ibebuchi [31]. Solid waste that was flooded into the water systems in various areas of the KwaZulu-Natal province accelerated water-borne diseases such as cholera due to Vibrio cholerae bacterium which became abundant in water resources [45-47].
Climate Variability in Southern Africa
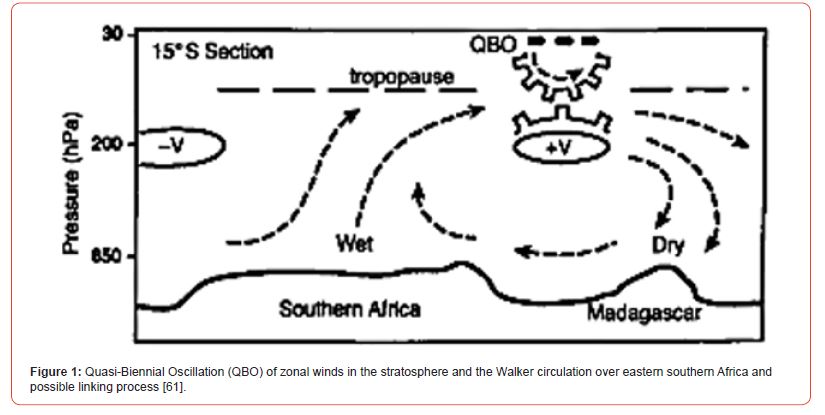
Climate variability in southern Africa has been widely acknowledged to present changes in rainfall and temperature patterns [48,49]. Climate variability associated with ENSO in tropical southern Africa is documented in such studies [50-52]. The ENSO phenomenon plays an important role in southern Africa’s climate variability through teleconnections between atmospheric and ocean conditions [53,54]. The warm events of ENSO are associated with drought in southern Africa, frequently and partly responsible for continental-scale and wider teleconnections [50, 55-57]. Previous studies by Chifurira and Chikobvu et al, Jury; Mamombe [55,58,59], indicated that the correlations between the Southern Oscillation Index (SOI) and rainfall decrease near the border between South Africa and Zimbabwe. Climate variability in southern Africa is also associated with the Indian Ocean Dipole (IOD) [54,60] and Quasi-biennial Oscillation (QBO) [61,62]. These are climate variability modes occurring naturally affecting surface temperature, atmospheric circulation, and precipitation [63-65]. When the stratospheric QBO is westerly, the ENSO is associated with wet conditions over southern Africa (Figure 1) and diminished rainfall when easterly. Jury [55] showed that in 1991/92, the stratospheric QBO was easterly, and the drought during that summer period was associated with the warm phase of ENSO.
Climate Variability Impacts
Drought and floods are part of natural climate variability and significant hydro-meteorological hazards that affect many communities in southern Africa [49,51,66]. Gemeda and Sima [67] indicated that 60% of the southern Africa population is affected by climate variability. These communities are most vulnerable to the effects of climate variability as a result of their economic status, the limited institutional and technological capacity to adapt to environmental changes, and greater dependence on climatesensitive resource sectors like agriculture, for subsistence and commercial purposes, and water [39]. For example, in the year 2000, Mozambique was affected by floods caused by tropical cyclone Eline which resulted in the reduction of the rate of annual economic growth from 10 to 4%, death of 800 people polluted water resources and destroyed agricultural production [39]. Flooding commonly pollutes water resources and destroys sanitation and drainage systems due to the debris that flows in the water systems [68,69]. According to IPCC (2021) [9] projections, increased flood risk will result in an additional 48 000 deaths of children under the age of 15 in 2030 in sub-Saharan Africa due to diarrhea and 33 000 deaths by 2050. Water stress caused by dry events are well documented. For example, severe droughts of 1992/93 in the KZN province of South Africa are detailed in Dube and Jury [14], and Ndlovu and Demlie [37] accounts for the 2015/16 drought. These events were associated with the El Niño phase of the ENSO and severely affected water quantity and quality. During these droughts, water resources were noted to have been reduced below 50% of their normal levels in large dams of the province such as Pongolapoort, Howick fall, Midmar and Goedertrouw Dam.
Drivers of Rainfall Variability Over Southern Africa
The position of southern Africa in relation to the tropical, subtropical, and mid-latitude pressure regimes is the essential factor affecting the subcontinental climate [70]. The thermallyindirect Ferrel and thermally-direct Hadley cells both descend in the subtropics, producing the subtropical quasi-stationary highpressure belt. The South Atlantic Anticyclone (centred around St. Helena) and South Indian Anticyclone (centered around Mauritius) are a dominant feature of southern Africa’s synoptic system, influencing rainfall patterns. Both have a well-defined annual meridional and zonal shift in their positions, moving 6⁰ northward and southward in winter and summer, respectively [62]. Most of South Africa receives rainfall from November to March, except the Western Cape which is a winter-rainfall region. The main synoptic systems that produce rainfall in South Africa include the ridging anticyclones in the south which account for between 16 to 25% of rainfall variation over the area [14,62], tropical-temperate troughs (TTTs) (30 to 60%) [71], cloud bands (48%), Mesoscale Convective Systems (MCSs) (14%), cut-off lows (COLs) (10%), and tropical and extra-tropical cyclones (28%) [19,72,73].
Floods occurring in different parts of South Africa might be due to wave cyclones from the southwest Atlantic Ocean and the Cold Benguela Channel [72], and cloud bands that are slow-moving [71,74]. Separated and storm line thunderstorms, mesoscale complexes and convective systems [75], and inland tropical lows (i.e., thermal) [76] also produce heavy rainfall and flooding. Cloud bands develop on the tropical-temperate troughs (TTTs) extending from the tropics to the mid-latitudes and they therefore, produce seasonal rainfall over the South Africa [71]. Jury [55,77] reported that TTTs contribute about 30% of the spring to summer rainfall totals, 60% of the January total, and 39% of the average annual total. This makes them crucial summer rainfall contributors over the inland areas. Heavy rainfall is also caused by slow-moving cloud bands as they pass through the Mozambique Channel and eastward across the Limpopo province of South Africa, and Madagascar.
Regional Inter-Annual Variability of Rainfall
Inter-annual rainfall variability over southern Africa is welldocumented [78-82]. An historical inter-annual rainfall variability analysis by Díaz and Vera using land stations and oceanic data at a 5° x 5° grid resolution in southern Africa to study the large-scale rainfall between 1979 and 1993, revealed that there was less mean rainfall per month from 1979 to 1993 as compared to 1951 to 1970. Between 1979 and 1993, there was an anomaly in the global rainfall patterns between the ocean and the land. For the tropical regions, the correlation coefficient between the yearly rainfall on land and ocean was determined to be -0.74. The correlation coefficient for the DJF season was determined to be -0.63 on a global scale. It was determined that greater anticyclonic (dryer) conditions over land are linked to increase precipitation over water. On the other hand, less precipitation over ocean regions tends to be linked to stronger cyclonic conditions in land areas. It was determined that the ENSO was associated with the first principal component mode (5.6%) of the rainfall (1979 to 1993) in the winter and summer seasons (r = 0.42). Between Central Indian Ocean and southern Africa, the first mode’s spatial loadings showed a weak dipole (0°-20°S, 50°E-I00°E). Gershunov and Michaelsen (1996) used monthly rainfall data from microwave-sounding units collected over a 15-year period to analyze the inter-annual variations in tropical ocean (30°N–30°S) rainfall. Utilizing a 2.5° x 2.5° grid resolution, they looked at 185 months between January 1979 and May 1994 and discovered that QBO and ENSO controlled the interannual variability.
Previous studies have looked at variations in rainfall in time and space at the continental scale [83-85]. For example, Nicholson [84] used monthly rainfall data for the years 1901–1973, to classify six geographical anomaly type of precipitation variability across Africa. The anomaly modes revealed two favored patterns: either anomalies of the opposite sign in the tropical against subtropical latitudes, or anomalies of the same sign throughout the continent. Significant peaks were observed at 2.2-2.4 and 3.3-3.8 years over Angola, east Africa, and southern Africa in the temporal characteristics of the southern Africa region. In the 2.2–2.4-time, strong coherence was observed with ENSO over the regions east of 30°E in southern and equatorial east Africa. In the 5.0–6.3-year oscillation, a substantial correlation with ENSO was observed over Angola, Malawi, South Africa, eastern Botswana, and southern Zambia.
Janowiak [83] used similar data as Nicholson [84] for annual and seasonal rainfall over Africa for the years 1927-1973. Three areas of action were identified by the higher modes of annual rainfall, which were found in southern Africa (7.9%), west Africa (12%), and east Africa (9.2%), using non-normalized data. The results showed that rainfall anomalies in the subcontinent’s equatorial and subtropical regions were out of phase. Three main areas of variability were identified by the principal combined analysis of December, January, February, and March (DJFM). Large positive loadings across southern Africa and weak negative loadings over central and east Africa (10°S-0°) were shown by the first mode (14.5%) (Nicholson, 1986). Large positive loadings over east Africa and modest negative loadings over southern Africa were observed in the second mode (13.3%). With the most positive loadings across west Africa, the third mode (11.3%) suggested that the loadings may alternate along a meridional axis. It was found that during ENSO events, the DJFM rainfall tends to be 10% to 25% below normal over the region east of 20°E and between 15°S and 30°S, but above normal east of 20°E between the equator and 10°S [84].
Inter-annual variability of rainfall over South Africa
Ndlovu et al., [51] examined the anomalies of rainfall in South Africa south of 25°S-30°S utilizing data station data from 1968– 2017. The study determined that for most years of the study period, the rainfall was below normal, and a decreasing trend was observed. Kruger [86] used rainfall annually station data (1910–2004) and applied principal component analysis (PCA). The findings indicated that thirty-two percent (32%) of the first mode had the highest loading over South Africa, and the time score had 10–13 year and biennial oscillations and the fourteen percent (14%) of the second mode had large loadings on the north of South Africa. It has been discovered that the inter-annual variability of rainfall in southern Africa is both spatially consistent and quasi-periodic. The ENSO and the South Indian Ocean dipole (SIOD) are the main contributors to inter-annual variability in South Africa. Tfwala et al. [23], studied the dynamics of droughts and inter-annual rainfall variability from 1918 to 2014 in South Africa. Long-term rainfall data from three stations from 1918 to 2014, and calculated Standardized Precipitation Index (SPI), were used. The results revealed that since the 1990s, dry events in South Africa were moderate, this was indicated by SPI values between -1.03 and –1.46, notably with year 1992 where the drought was proven by many studies to be severe (SPI ≤ -2) [23]. Similar results were observed in Dube and Jury [14]; Ibebuchi [31]; Mashao et al. [87]; Ndlovu and Demlie [37]; Ndlovu et al. [51] studies where it was observed that 1991/92 drought was the worst drought in South Africa because of the impacts that were experienced in the region. The declining trends of rainfall were observed for the period of 1983–2010 in South Africa. Decreasing annual rainfall patterns were observed by Parida and Moalafhi [88] from 1981. Nel [89], observed that the rainfall trends in the KZN Drakensberg region are becoming more variable. Other studies detailing inter-annual rainfall variability are based on trends and time series analyses [90-94].
Cut-off Lows (COLs)
A cut-off low (COL) is a synoptic scale baroclinic system known for its stormy weather, commonly causing heavy rainfalls and floods [73,95]. COLs are formed and developed over the midlatitudes, tropospheric polar jet stream equatorial side resulting in closed cyclones in the middle and upper troposphere. According to Dube and Jury [14], COLs are a severe westerly trough form which account for many flood-producing rains observed in South Africa, such as the 1987 KZN floods. The frequency of occurrence of COLs exhibits a semi-annual oscillation with maxima in March to May and September to November, i.e. an austral autumn maximum and a secondary peak in spring, respectively [62]. COLs can occur all-year-round across South Africa [87,96,97]. The east and south coasts, as well as inland areas, are frequently impacted (Favre et al., 2013). Deep moist convection is linked to COLs, which frequently result in prolonged, intense rainfall that can cause floods [96,98]. However, COLs are more common in the southern regions of South Africa and much less common in the far north. Nevertheless, it has also been discovered that COLs account for almost 10% of the extreme rainfall events that occur in South Africa’s northeast [19]. According to Masayo et al. and Molekwa et al. [99], there is an average yearly frequency of eleven (11) COLs over southern African region. Favre et al. [100] observed that 25 to 35% annual rainfall over the eastern, central and southern South Africa is driven by COLs. The cold periods of the ENSO, which coincide with aboveaverage rainfall occurrences in southern Africa, also have an impact on the incidence of COLs [99]. Despite their brief duration, these systems have the potential to be extremely intense, which increases their contribution to South Africa’s mean rainfall, or nearly 25–35% of the country’s yearly rainfall [100]. Multiple low-level circulation patterns can be associated with COLs; however, a strong anticyclone ridge in the low-levels poleward of the upper COL is a common feature of COLs, [101,102] observed that riding anticyclone cooccurring with COLs and TTTs are associated with 16% of the total annual rainfall in South Africa.
Ridging anticyclones
Heavy rainfall occurs over South Africa as a result of a ridging anticyclone [103]. Ridging anticyclones at the surface serve as the dynamical trigger for surface convergence [87]. Jury [104] studied the meteorology of ridging anticyclones on the KZN coastal regions utilizing Principal Component Analysis of daily ERA5 sea level pressure (SLP) from 1980 to 2021. Ridging highs that deliver the coast of KZN beneficial October-December rainfall are thus dependent on downstream climatic coupling with the Indian Ocean Dipole. Ridging anticyclones are essential to rainfall occurrences and are vital to South Africa’s moisture budget since they are the primary mechanism that enables the movement of moisture from the Southwest Indian Ocean [105,106]. According to Engelbrecht et al. [102] and Ndarana et al. [106], ridging anticyclones account for 46% of yearly rainfall in the all-year rainfall region and 60% of summer rainfall days in the summer rainfall over southern Africa. Due to the ongoing, intense rainfall that is linked with these systems, which frequently causes floods, the destruction of vital infrastructure, and the loss of lives and livelihoods, they have had numerous effects on water resources in South Africa [99]. The eastern coast seaboard of South Africa, was impacted hard by heavy rains in April 2019 that resulted in disastrous floods that killed more than 85 people, more than 1400 people were displaced and severely damaged infrastructure, including hospitals [97]. An operational forecaster’s synoptic map analysis at the South African Weather Service (SAWS) indicated that a COL pressure system connected to a ridging Atlantic Ocean anticyclone was the cause of this extreme rainfall event [97]. Crimp and Mason [107] observed that the severe rainfall seen in eastern South Africa in February 1996 was caused by a tropical low in association with a ridging anticyclone. For instance, over 100 mm of rain was recorded in the coastal regions, while over 90 mm was recorded in the interior over the north-eastern region of South Africa.
Droughts
Drought is a serious worldwide problem associated with extreme weather phenomena that unfold gradually and insidiously when compared to other natural disasters [108]. Drought occurs as a result of prolonged lack of rain over an extended period of time [109]. Essentially, it is the extent of dryness when compared to average rainfall for a specific area and the how long the dry period lasts [110]. Sometimes drought can be confused with water scarcity, aridity, and desertification. Unlike aridity, drought is not a permanent climate condition that can be worsened by high temperatures, strong winds, and low humidity [111]. All types of droughts, whether lasting days, seasons, or years, have been associated with prevailing anticyclone conditions, which majorly influence the weather and climate in southern Africa [62]. In South Africa, drought usually follows a period of lower-than-average rainfall following an El Niño event [112]. Most droughts in South Africa, since the late 1960s, have been associated with El Niño events [113].
Droughts have an influence on a nation’s social, environmental, and economic well-being, assert [114]. A socio-economic drought can follow the onset of meteorological, hydrological, and agricultural droughts. Demand for economic commodities can exceed supply during socioeconomic declines. Water supply constraints brought on by climate variability and change might be the source of this [115]. Droughts have impact on society such as causing famine, unemployment, income reductions, poverty, and a lack of water supplies [116]. For instance, the 1992 worst drought in South Africa resulted in the loss of about 50 000 jobs in the agricultural sector and 20 000 formal sectors [117]. Pathack et al., Jury, and Dube and Jury [118-120] have developed conceptual frameworks for dry (and wet) summer scenarios over southern Africa from observations of the circulation systems during these periods. Droughts disturb the environment in several ways such as crops loss, soil erosion acceleration, pollution increases, biodiversity, water-borne diseases, and fire hazards due to dry vegetation [121].
Botai et al. [122] studied droughts characteristics in South Africa in the Free State (FS) and North West (NW) Provinces since these area are vulnerable to drought with the effects on agricultural production and water quantity. Due to major water reserves and protected agricultural production, both provinces are essential to the South African economy. As a result, the study was conducted to examine how the drought has changed historically in the FS and NW Provinces from 1985 to 2015. To investigate and characterize variations in drought duration, intensity, frequency, and severity in the FS and NW Provinces between 1985 and 2015, the Standardized Precipitation Evapotranspiration Index (SPEI) and Standardized Precipitation Index (SPI), were calculated based on monthly meteorological data from 14 meteorological stations within the FS and NW Provinces. The findings demonstrated that throughout the chosen stations, there were negative and positive trends. Specifically, over 60% of the meteorological station showed a decreasing trend in the FS Province, compared to NW Province. The FS been suffering and experiencing the increasing dryness during the study period which causes the decrease in water resources and agricultural productions. The examination of the drought evaluation indicators (DEIs) derived from the SPEI showed that between 1985 and 2015, the intensity of the drought was greater in NW Province and more noticeable in FS in terms of both frequency and severity.
Hisdal and Tallaksen [123] estimated the characteristics of regional hydrological and meteorological drought in Denmark (Northern Europe). The study introduced the techniques of calculating the probability of a particular region to be impacted by a drought and demonstrates the potential of calculating the characteristics of hydrological and meteorological drought. The procedures of the calculations were applied to monthly rainfall and streamflow series individually, which were then continuously modified by the Empirical Orthogonal Functions (EOF) technique. The 260 14 x 17 km grid-cells that make up Denmark were used to interpolate the monthly average and the EOF-weight coefficients using kriging. After that, the frequency distributions of the first two amplitude functions (streamflow) or the three (rainfall) were calculated. Streamflow and rainfall data analysis has been widely utilized to study the characteristics of drought [124-126]. The findings indicated that in Denmark, meteorological droughts are more frequent and cover larger regions than hydrological droughts. Hence, the probability revealed that if there is meteorological drought, the entire Denmark will be covered. Rainfall-related droughts are less pronounced in Denmark than meteorological droughts. They usually happen later than meteorological droughts and result from a combination of factors including inadequate rainfall, insufficient storage conditions, and high evaporation losses.
Temperature Variability
Studies such as by Kruger and Shongwe [76], Hughes and Balling [127], Warburton Schulze [128] and Unganai [129] have detailed temperature changes over southern Africa. Based on such studies, the general conclusion is that minimum and maximum temperatures are increasing at different rates. Minimum temperatures are increasing faster than maximum temperatures. Thus, a warming trend has been the overall outcome. The hydrological cycle usually intensifies in response to temperature increases because of an increase in both rainfall and evaporation [2]. Variations in temperature can have an impact on soil moisture, groundwater reserves, rainfall patterns, and the frequency of floods and droughts [130]. Therefore, variations in temperature have a direct impact on the availability of water resources.
Kruger and Sekele [131] observed an increase in warm and cold extremes in South Africa in their analysis. An earlier study by Muhlenbruch-Tegen [132] found no evidence to conclude that South Africa was cooling or warming in a period 1940 to 1989. Levey [133] reported an upward trend of 10% (+ 1.5°C) in the winter series. Jones et al. [134] uncovered a 0.31°C per decade warming rate. At the same time, Karl et al. [135] concluded that there was an increase in both minimum and maximum temperatures. A study by Tshiala et al. [136] in Limpopo, South Africa, revealed a mean annual temperature increase of 0.12°C per decade. At hemispheric level, Collins [137], reported that strong trends of rising temperatures were observed in the Southern Hemisphere of Africa. According to Morishima and Akasaka [138], the yearly mean surface temperature in southern Africa is on the rise throughout the continent, with Namibia and Angola experiencing very rapid increases. The Southern African Development Community (SADC) region’s daily (minimum and maximum) temperatures were analyzed by Kusangaya et al. [2]. It was concluded that the extremes of temperature exhibit patterns consistent with warming over the majority of the region, with a consistent increase in the diurnal temperature range (DTR) across Botswana, Namibia, Mozambique and Zambia. These increases coincided with significant increase in maximum temperature extremes than minimum temperature extremes. As reported by Hulme et al. [139], temperatures in southern Africa were between 0.2 and 0.3°C warmer than the average for the period 1961–1990. These temperatures were higher in the 1990s than they were earlier in the century considering that temperatures are rising throughout southern Africa, with minimum temperatures increasing quicker than maximum temperatures [140]. Consequently, there is also a discernible rise in warm extremes and a fall in cold extremes. Apart from the overall trend of rising temperatures, there are still unanswered questions regarding the variation in change magnitude across the region.
Water Resources
Climate variability is accompanied by fluctuations in temperature and rainfall trends. According to Nsubuga et al. [52], the rate of evaporation is commonly affected by the temperature increases on earth which bears a huge effect on water resources. Since the 1960s, decreases in annual rainfall were observed in some African countries [141-143]. According to Fauchereau et al. [144], annual climate variability has the impacts on ground and surface water resources and related activities such as tourism, production of agriculture, and hydropower, since it is associated with heavy rainfalls and drought, seasonal changes and extreme weather events in the African continent. In southern Africa, water resources are susceptible to the variations and changes in climate due to fluctuations and volatility of dams and lake volumes [145-146]. Surface runoff is noted to be the essential parameter contributing to water levels in the dams and lakes [147,148]. According to the IPCC (2021) [9], in the future, with the effects of global warming, droughts and floods will occur more frequently and more severely over the earth, and the fluctuations of precipitation patterns and surface water flow will worsen. Similar studies elsewhere in the world, such as in South Korea, have shown that El Niño, La Niña, climate change, and variability are responsible for seasonal variability in precipitation and runoff intensifies [149-151]. Ofori et al. [152] and Mwadzingeni et al. [153], have predicted that drought frequency and intensity will increase in future in Sub-Saharan Africa (SSA) because of the changes and variations in climate which will negatively affect water resources.
Projections of inflows under variations in climate: Southern Africa
A number of studies have provided multiple runoff forecasts under the changing climate in the southern Africa region. In the dry tropics, river runoff and water availability are projected to decline by 10% to 30% (IPCC, 2007) [154] by the 2050. Due to more evaporation and less rainfall, Arnell [4] predicted that the Zambezi River source will experience a 26 to 40% decrease in runoff in the future since an increase in evaporative capacity may cause reservoir outflows to decrease [4]. Furthermore, low storage episodes frequency is expected to increase due to the projected increased frequency of future droughts. This will undoubtedly have an impact on future hydropower generation from dams like the Cabora Bassa and Kariba [7]. Taylor et al. (2013), demonstrated that the changes in climate will result in a 40% or greater decline in surface and subsurface runoff in the future Zambezi basin in Mozambique.
Yamba et al. [7] demonstrated that as a result of drought frequency increases, reducing run-off and, consequently, reservoir storage capacity, the Zambezi Basin’s potential to generate hydroelectric power will gradually decline for both current and proposed hydroelectric power schemes. Most studies have generally concluded that streamflow is anticipated to decrease by 2050. For instance, Beck and Bernauer [155] predicted that streamflow in the Zambezi basin would decline by up to 20% in 2050, while Matondo [156] predicted that it would decrease by up to 40% in Eswatini (Swaziland) by 2060. Catchment decreases in southern Africa are projected by 2050 as follows: Pungwe catchment (shared between Mozambique and Zimbabwe) decrease of up to 75% [2,157]; Limpopo catchment (South Africa) by up to 35% [158]; Thukela catchment area (South Africa) up to 18%, the Okavango (Botswana) by up to 20% [63]; the Zambezi, Ruvhuma, Limpopo and Botswana Orange catchments by up to 45% [4]; and the Gwayi, Sebakwe, and Odzi, catchments (Zimbabwe) by up to 50% [159]. Nonetheless, increases in streamflow of between 16 and 38% are predicted for some catchments, such as portions of the Thukela catchment area [160,161]. Additionally, according to models developed by Masocha et al. [162] and Mhlanga-Ndlovu et al. [163], several catchment areas in Eswatini (Swaziland) are expected to have increased streamflow of between 5% and 34%. Despite the majority for studies mentioned above concluding that streamflow will drop, there is still no consent regarding the exact amount of the decrease or rise [164,168]. Further data can be obtained by using distributed hydrological models and downscaled General Circulation Models (GCMs), particularly when modelling changes in streamflow under climate variability and change, in order to lessen this uncertainty. Additionally, improved projected streamflow changes for southern Africa are probably to come from the use of an assembly of climate models.
Conclusion
This paper has presented a review of literature on climate variability and change over southern Africa, with special emphasis on South Africa. Significant rainfall-producing meteorological systems, such as COLs, TTTs, and ridging anticyclones were presented. Various modes of climate variability over the region were presented. Emphasis was placed on projections relating to climate and water resources in southern Africa. The review of climate variability and its impacts on water resources demonstrates the significant and complex relationship between the two. The region’s diverse climate and topography contribute to a wide range of climate and weather conditions, which in turn have notable impacts on water availability and quality. Understanding and addressing these impacts are crucial for ensuring the sustainable management of water resources. Despite the fact that so much work has been done in the subject under review in this paper, there are still many unresolved issues. While there is a general consensus in studies on climate variability and change in the region, uncertainties still exist with regard to the rate of changes in temperature, rainfall patterns under a changing climate remain patchy and incoherent, and knowledge gaps exist too with regards to catchment changes.
Acknowledgement
This work is part of a broader research project. The University of KwaZulu-Natal is thanked for the time and resources made available to undertake it.
References
- Cucalon LG, King G, Kaseer F, Arambula Mercado E, Martin AE, et al. (2017) Compatibility of recycled binder blends with recycling agents: rheological and physicochemical evaluation of rejuvenation and aging processes. Ind Eng Chem Res 56 (29): 8375-8384.
- Brown ER, Kandhal PS, Roberts FL, Kim YR, Lee D-Y, et al. (2009) Hot mix asphalt materials, mixture design, and construction, Third Edit: NAPA Research and Education Foundation, Lanham, Maryland, USA.
- Deef-Allah E, Abdelrahman M (2021) Investigating the relationship between the fatigue cracking resistance and thermal characteristics of asphalt binders extracted from field mixes containing recycled materials. Transp Eng 4.
- Petersen JC (2009) A review of the fundamentals of asphalt oxidation chemical, physicochemical, physical property, and durability relationships, Transportation Research Circular E-C140, Washington, DC, USA.
- Sreeram A, Leng Z (2019) Variability of RAP binder mobilisation in hot mix asphalt mixtures. Constr Build Mater 201: 502-509.
- Liang M, Xin X, Fan W, Zhang J, Jiang H, et al. (2021) Comparison of rheological properties and compatibility of asphalt modified with various polyethylene. Int J Pavement Eng 22 (1): 11-20.
- Sreeram A, Leng Z, Hajj R, Bhasin A (2019) Characterization of compatibility between aged and unaged binders in bituminous mixtures through an extended HSP model of solubility. Fuel 254: 115578.
- McDaniel RS, Soleymani H, Turner P, Peterson R (2000) Recommended use of reclaimed asphalt pavement in the Superpave mix design method. Technical Report, NCHRP D9-12.
- Sreeram A, Leng Z, Zhang Y, Padhan RK (2018) Evaluation of RAP binder mobilisation and blending efficiency in bituminous mixtures: An approach using ATR-FTIR and artificial aggregate. Constr Build Mater 179: 245-253.
- Zhao S, Huang B, Shu X, Moore J, Bowers B (2016) Effects of WMA technologies on asphalt binder blending. J Mater Civ Eng 28 (2): 04015106.
- Noferini L, Simone A, Sangiorgi C, Mazzotta F (2017) Investigation on performances of asphalt mixtures made with reclaimed asphalt pavement: Effects of interaction between virgin and RAP bitumen. Int J Pavement Res Technol 10(4): 322-332.
- Deef-Allah E, Abdelrahman M (2022) Interactions between RAP and virgin asphalt binders in field, plant, and lab mixes. World J Adv Res Rev 13(1): 231-249.
- Deef-Allah E, Abdelrahman M, Ragab M (2022) Components’ exchanges between recycled materials and asphalt binders in asphalt mixes. Adv Civ Eng Mater 11(1): 20210105.
- Ding Y, Huang B, Shu X, Zhang Y, Woods ME (2016) Use of molecular dynamics to investigate diffusion between virgin and aged asphalt binders. Fuel 174: 267-273.
- Dong F, Yu X, Chen J, Liu S, Chen Q (2017) Investigation on compatibility and microstructure of PCBs-modified asphalt. J Appl Polym Sci 134 (25).
- Becker Y, Müller AJ, and Rodriguez Y (2003) Use of rheological compatibility criteria to study SBS modified asphalts. J Appl Polym Sci 90 (7): 1772-1782.
- Airey GD (1997) Rheological characteristics of polymer modified and aged binder. Ph.D. dissertation, Univ. Nottingham, USA.
- Ashish PK, Singh D, Bohm S (2017) Investigation on influence of nanoclay addition on rheological performance of asphalt binder. Road Mater. Pavement Des 18(5): 1007-1026.
- Ait-Kadi A, Brahimi B, Bousmina M (1996) Polymer blends for enhanced asphalt binders. Polym Eng Sci 36(12): 1724-1733.
- Liang M, Liang P, Fan W, Qian C, Xin X, et al. (2015) Thermo-rheological behavior and compatibility of modified asphalt with various styrene-butadiene structures in SBS copolymers. Mater Des 88: 177-185.
- Ajji A and Utracki LA (1996) Interphase and compatibilization of polymer blends. Polym Eng Sci 36 (12): 1574-1585.
- Gaestel C, Smadja R, Lamminan K (1971) Contribution to knowledge of the properties of road bitumens. General Review of Roads and Aerodromes. 466: 85-92.
- Oliver JWH (2009) Changes in the chemical composition of Australian bitumens. Road Mater. Pavement Des 10(3): 569-586
- Oyekunle LO (2007) Influence of chemical composition on the physical characteristics of paving asphalts. Pet Sci Technol 25 (11): 1401-1411.
- ASTM D2172 / D2172M-17e1 (2017) Standard Test Methods for Quantitative Extraction of Asphalt Binder from Asphalt Mixtures. West Conshohocken, PA: ASTM International.
- ASTM D5404 / D5404M-12 (2017) Standard Practice for Recovery of Asphalt from Solution Using the Rotary Evaporator. West Conshohocken, PA: ASTM International.
- ASTM D2872-19 (2019) Standard Test Method for Effect of Heat and Air on a Moving Film of Asphalt (Rolling Thin-Film Oven Test). West Conshohocken, PA: ASTM International.
- ASTM D7175-15 (2015) Standard Test Method for Determining the Rheological Properties of Asphalt Binder Using a Dynamic Shear Rheometer. West Conshohocken, PA: ASTM International.
- ASTM E1131-20 (2020) Standard Test Method for Compositional Analysis by Thermogravimetry. West Conshohocken, PA: ASTM International.
- Jiménez-Mateos JM, Quintero LC, Rial C (1996) Characterization of petroleum bitumens and their fractions by thermogravimetric analysis and differential scanning calorimetry. Fuel 75 (15): 1691-1700.
- ISO 11358-1 (2014) Plastics-Thermogravimetry (TG) of Polymers- Part 1: General Principles. International Organization for Standardization. First edit.
- Holleran G, Holleran I (2010) Bitumen chemistry using cheaper sources - an improved method of measurement by TLCFID and the characterisation of bitumen by rheological and compositional means. in 24th ARRB Conf., Melbourne, Australia.
- Jiang C, Larter SR, Noke KJ, Snowdon LR (2008) TLC-FID (Iatroscan) analysis of heavy oil and tar sand samples. Org Geochem 39(8): 1210-1214.
- Wang J, Wang T, Hou X, Xiao F (2019) Modelling of rheological and chemical properties of asphalt binder considering SARA fraction. Fuel 238(4): 320-330.
- Ashoori S, Sharifi M, Masoumi M, Salehi MM (2017) The relationship between SARA fractions and crude oil stability. Egypt. J Pet 26 (1): 209-213.
- Xiong R, Guo J, Kiyingi W, Feng H, Sun T, et al. (2020) Method for judging the stability of asphaltenes in crude oil. ACS Omega 5(34): 21420-21427.
- Paliukaitė M, Vaitkus A, Zofka A (2014) Evaluation of bitumen fractional composition depending on the crude oil type and production technology. in the 9th Int. Conf. “ENVIRON. ENG.”, Vilnius, Lithuania.
- Xu G, Gong X, Yu Y, Chen X (2021) A rapid method for the determination of SBS content based on the principle of orthogonal testing. Appl Sci 11 (22): 10911.
- Ye ZC, Chen D, Ling C, Guan FY (2014) Quantifying SBS content in modified asphalt using Fourier transform infrared spectroscopy. Adv Mat Res 1065-1069: 691-695.
- Davis J (2024) Modified asphalt strengthens roofing shingles. The Magazine of the Asphalt Institute.
- Nazzal MD, Kim SS, Abbas A, Qtaish LA, Holcombe E, et al. (2009) Fundamental evaluation of the interaction between RAS/RAP and virgin asphalt binders. Technical Report, Ohio DOT, FHWA/OH-2017-24.
- Alvergue A, Elseifi M, Mohammad LN, Cooper SB, Cooper S (2014) Laboratory evaluation of asphalt mixtures with reclaimed asphalt shingle prepared using the wet process. Road Mater. Pavement Des 15 (sup1): 62-77.
- Buttlar WG, Abdelrahman M, Majidifard H, Deef-Allah E (2021) Understanding and improving heterogeneous, modern recycled asphalt mixes. Technical Report, Missouri DOT, cmr 21-007.
- Deef-Allah E, M Abdelrahman (2022) Characterization of asphalt binders extracted from field mixtures containing RAP and/or RAS. World J Adv Res Rev 13 (1): 140-152.
- Guo W, Guo X, Chang M, Dai W (2018) Evaluating the effect of hydrophobic nanosilica on the viscoelasticity property of asphalt and asphalt mixture. Materials 11 (11): 2328.
- Deef-Allah E, Abdelrahman M, Fitch M, Ragab M, Bose M, et al. (2019) Balancing the performance and environmental concerns of used motor oil as rejuvenator in asphalt mixes. Recycling 4 (1).
- Deef-Allah E, Abdelrahman M, Hemida A (2020) Improving asphalt binder’s elasticity through controlling the interaction parameters between CRM and asphalt binder. Adv Civ Eng Mater 9 (1): 262-282.
- Li J, Huang X, Zhang Y, Xu M (2009) Bitumen colloidal and structural stability characterization. Road Mater Pavement Des 10 (sup1): 45-59.
- Mansoori GA (1997) Modeling of asphaltene and other heavy organic depositions. J Pet Sci Eng 17 (1-2): 101-111.
- Speight JG (2015) Asphaltene deposition. in Asphalt Materials Science and Technology, Oxford, UK, Butterworth-Heinemann pp. 303-337.
- Zhang D, Chen M, Wu S, Liu J, Amirkhanian S (2017) Analysis of the relationships between waste cooking oil qualities and rejuvenated asphalt properties. Materials 10 (5): 508.
- Petersen JC (2009) A review of the fundamentals of asphalt oxidation: chemical, physicochemical, physical property. in Transp. E-Circular E-C140, Transp. Res. Board, Washington, DC, USA.
- Schlepp L, Elie M, Landais P, Romero MA (2001) Pyrolysis of asphalt in the presence and absence of water. Fuel Process. Technol 74 (2): 107-123.
- Zhang C, Xu T, Shi H, Wang L (2015) Physicochemical and pyrolysis properties of SARA fractions separated from asphalt binder. J Therm Anal Calorim 122 (1): 241-249.
- Radhakrishnan CK, Sujith A, Unnikrishnan G (2007) Thermal behaviour of styrene butadiene rubber/poly (ethylene-co-vinyl acetate) blends. J Therm Anal Calorim 90 (1): 191-199.
- Mothé MG, Leite LFM, Mothé CG (2011) Kinetic parameters of different asphalt binders by thermal analysis. J Therm Anal Calorim 106 (3): 679-684.
- Jing-Song G, Wei-Biao F, Bei Jing Z (2003) A study on the pyrolysis of asphalt. Fuel 82 (1): 49-52.
- Puello J, Afanasjeva N, Alvarez M (2013) Thermal properties and chemical composition of bituminous materials exposed to accelerated ageing. Road Mater. Pavement Des 14 (2): 278-288.
-
Sibongakonke BH Hadebe and Lawrence T Dube*. Climate Variability over Southern Africa and Implications for Water Resources: A Review. Adv in Hydro & Meteorol. 1(4): 2024. AHM.MS.ID.000517.
-
Climate variability and change; ENSO; drought; floods; temperature; rainfall
-
This work is licensed under a Creative Commons Attribution-NonCommercial 4.0 International License.